the Creative Commons Attribution 4.0 License.
the Creative Commons Attribution 4.0 License.
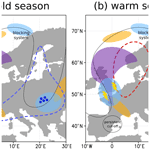
Atmospheric blocking and weather extremes over the Euro-Atlantic sector – a review
Lisa-Ann Kautz
Olivia Martius
Stephan Pfahl
Joaquim G. Pinto
Alexandre M. Ramos
Pedro M. Sousa
Tim Woollings
The physical understanding and timely prediction of extreme weather events are of enormous importance to society due to their associated impacts. In this article, we highlight several types of weather extremes occurring in Europe in connection with a particular atmospheric flow pattern, known as atmospheric blocking. This flow pattern effectively blocks the prevailing westerly large-scale atmospheric flow, resulting in changing flow anomalies in the vicinity of the blocking system and persistent conditions in the immediate region of its occurrence. Blocking systems are long-lasting, quasi-stationary and self-sustaining systems that occur frequently over certain regions. Their presence and characteristics have an impact on the predictability of weather extremes and can thus be used as potential indicators. The phasing between the surface and the upper-level blocking anomalies is of major importance for the development of the extreme event. In summer, heat waves and droughts form below the blocking anticyclone primarily via large-scale subsidence that leads to cloud-free skies and, thus, persistent shortwave radiative warming of the ground. In winter, cold waves that occur during atmospheric blocking are normally observed downstream or south of these systems. Here, meridional advection of cold air masses from higher latitudes plays a decisive role. Depending on their location, blocking systems also may lead to a shift in the storm track, which influences the occurrence of wind and precipitation anomalies. Due to these multifaceted linkages, compound events are often observed in conjunction with blocking conditions. In addition to the aforementioned relations, the predictability of extreme events associated with blocking and links to climate change are assessed. Finally, current knowledge gaps and pertinent research perspectives for the future are discussed.
Weather extremes have a great significance for society, as they pose a threat to human life and can result in enormous economic damage and disruption. In Europe, heat waves are among the deadliest natural hazards, while storms and flooding events are among the costliest (Kovats and Kristie, 2006; Mohleji and Pielke, 2014; Raška, 2015; Forzieri et al., 2017). The heat wave in 2010, which affected eastern Europe and large parts of Russia, is a prominent example of such an event (e.g., Grumm, 2011). Heat records were broken in many areas, and Moscow recorded temperatures of almost 40 ∘C (Barriopedro et al., 2011). The heat wave was associated with an extreme drought resulting in thousands of forest fires that damaged agriculture (Witte et al., 2011). The forest fires also caused air pollution associated with health risk. Another example for a high-impact weather event is the cold spell at the beginning of 2012 that affected Europe (de'Donato et al., 2013; Demirtaş, 2017). Temperatures around −40 ∘C were observed in Russia and parts of Scandinavia, but also in southern European countries like Greece, temperatures fell below −20 ∘C. In addition to the low temperatures, parts of southeastern Europe also experienced heavy snowfall, which strongly affected the transport sector (Davolio et al., 2015). In total, 650 deaths are attributed to this cold spell (DWD, 2012). Besides cold and heat waves, Europe is affected by other types of high-impact weather events, like floods. In autumn 2000, several heavy-precipitation events led to flooding in Switzerland and northern Italy (Lenggenhager et al., 2019). In Switzerland, basements and streets were flooded, and some roads had to be completely closed due to the danger of landslides (https://www.swissinfo.ch/eng/gondo-marks-tenth-anniversary-of-disaster/28532856, last access: 21 November 2021). As different as these examples for weather extreme events might have been, they have something in common. Namely the prevailing large-scale flow pattern in the troposphere, which was strongly influenced by atmospheric blocking (hereinafter referred to as blocking).
Blocking systems can be described as long-lasting, quasi-stationary and self-sustaining tropospheric flow patterns that are associated with a large meridional flow component and, thus, an interruption and/or deceleration of the zonal westerly flow in the midlatitudes (e.g., Liu, 1994; Nakamura and Huang, 2018). However, a strong zonal flow may be simultaneously found north and south of the blocking systems. Their onset and decay phases are characterized by transitions from a more zonal to a more meridional flow pattern and vice versa, which is challenging for forecast models (e.g., Frederiksen et al., 2004). In addition, blocking is associated with complex dynamics that link different spatial and temporal scales and affect both their internal evolution and interactions with the flow environment (e.g., Shutts, 1983; Lupo and Smith, 1995). Blocking systems extend vertically across the whole troposphere and are at the surface typically associated with large high-pressure systems (e.g., Schwierz et al., 2004), although the occurrence of heat lows below the blocking ridge is also observed. They occur both over the oceans and over land masses (e.g., Barriopedro et al., 2006; Tyrlis and Hoskins, 2008) and may cause extreme weather events, whereas the type of extreme event is sensitive to the exact location of the blocking system (e.g., Brunner et al., 2018). Moreover, different surface extremes at different locations (and sometimes at the same time) can be caused by the same blocking system (e.g., Lau and Kim, 2012).
Since the 1950s, the phenomenon of blocking has been studied by atmospheric scientists. Both the synoptic timescales (e.g., Colucci, 1985; Crum and Stevens, 1988) and the climate perspective (e.g., Nabizadeh et al., 2019) are of particular interest. Many studies are also available on the dynamical aspects (e.g., Berggren et al., 1949; Steinfeld and Pfahl, 2019), whereby interactions with different scales (e.g., Lupo and Smith, 1995; Luo et al., 2014) or with other flow features (e.g., Shutts, 1983; Shabbar et al., 2001) are considered. An important branch of research is concerned with how well blocking systems can be predicted (e.g., Bengtsson, 1981; Matsueda, 2009) or how blocking systems affect the quality of weather predictions (e.g., Quandt et al., 2017; Ferranti et al., 2018). A review on blocking, in particular with regard to the projected changes in blocking occurrence and characteristics under climate change, was provided by Woollings et al. (2018). A general review has recently been published by Lupo (2020). Although the range of studies dealing with blocking is wide, there is no summary yet that specifically addresses the influence of this phenomenon on surface weather extremes. This paper focuses on this gap. In doing so, we both highlight how specific these influences on each type of extreme can be and make a connection between them. In this context, the consideration of case studies is a central issue, as it can best illustrate the complexity and variability in the relationship between blocking and surface extremes.
The article is structured as follows. In Sect. 2, a brief summary of blocking characteristics addressing also important dynamical features as well as the predictability of blocking is provided. Section 3 deals with temperature extremes, while hydrological extremes are discussed in Sect. 4. For both types of extremes, an overview, a description of the relevant dynamics and several case studies are presented in both sections. Section 5 provides an overview on other extremes related to extreme winds and addresses compound events. In Sect. 6, the issue of predictability is revisited but with a focus on the impact of blocking on the predictions of surface extreme events. In Sect. 7, we address changes in blocking and weather extremes due to climate change. An outlook and pertinent research perspectives are presented in the last section.
2.1 Definition and characteristics
Blocking systems are characterized by their persistence, quasi-stationarity and self-preservation. Although these characteristics are common to most blocking systems, there is no unique definition owing to the rich diversity in synoptic structure. Following the pioneering study of Rex (1950), many consider an essential feature of blocking to be a sharp transition from a zonal to meridional flow pattern, as the jet is typically split into two branches around the system. Blocking systems generally fall into the following categories, examples of which are shown in Woollings et al. (2018).
-
Rex or dipole blocks consist of an anticyclone lying poleward of a cyclone. These are closely linked to the breaking of atmospheric Rossby waves which acts to reverse the usual meridional flow gradients (Hoskins et al., 1985; Pelly and Hoskins, 2003a). Wave breaking can take an anticyclonic or cyclonic form, and both lead to similar meridional dipole structures (Weijenborg et al., 2012; Masato et al., 2013a).
-
Omega blocks are characterized by a huge anticyclone flanked by an upstream and a downstream cyclone leading to an omega-shaped flow pattern (Ser-Giacomi et al., 2015). Although most common in the Pacific/North America sector, they do also occur over Eurasia.
-
Amplified ridges without any closed contours (in, e.g., 500 hPa geopotential height) are also able to block the zonal flow and to lead to a dominating meridional flow component, especially in summer (Sousa et al., 2018). They are more common at lower latitudes.
A large array of blocking indices has been developed, each designed to capture one or more of the structures within this diversity. One approach is to identify blocking as a long-lasting anomaly, for example associated with a negative potential vorticity (PV) anomaly at 320 K (Schwierz et al., 2004). Another way to identify blocking is to detect the reversal in meridional gradients, for example, in the 500 hPa geopotential height (Tibaldi and Molteni, 1990; Scherrer et al., 2006) or the potential temperature at 2 PVU (Pelly and Hoskins, 2003a). Some indices are one-dimensional (e.g., the index of Pelly and Hoskins, 2003a, is calculated along the so-called “central blocking latitude”), while others present blocking patterns as two-dimensional structure (Masato et al., 2013a). In some studies, additional spatial and temporal criteria addressing blocking duration (number of blocked days) and the extension of blocking systems are considered (Barnes et al., 2012). Please note that in some studies the indices mentioned are used in a modified form, which may lead to varying results (e.g., Schalge et al., 2011). A recent work by Sousa et al. (2021) has explored a conceptual model for the life cycle of blocks, considering the dynamical process of incipient subtropical ridges transitioning towards an Omega block, trough wave breaking and towards the mature phase of a fully secluded Rex block. A detailed overview of blocking detection indices is provided by Barriopedro et al. (2010). Besides these more synoptic descriptions (Liu, 1994), blocking can also be described by local finite-amplitude wave metrics (G. Chen et al., 2015; Huang and Nakamura, 2016; Martineau et al., 2017).
Blocking in the Northern Hemisphere occurs predominantly for specific regions (Barriopedro et al., 2006; Tyrlis and Hoskins, 2008), both over land and oceans. Over land, blocking is preferably found over a region reaching from Europe (especially over Scandinavia) (Tyrlis and Hoskins, 2008) to Asia (especially over the Ural Mountains) (Cheung et al., 2013). Europe is identified as a dominant region of blocking in most indices, due to the configuration of a strong, meridionally tilted storm track upstream of a large landmass. Blocking also occurs frequently over Greenland with strong downstream impacts on Europe associated with the negative phase of the North Atlantic Oscillation (NAO) (Davini et al., 2012). Additionally, blocking occurs over the northern United States and Canada, where it is also associated with extreme events, such as temperature or precipitation anomalies, for example, the Gulf of Alaska blocking event in summer 2004 leading to abnormally high temperatures and less-than-normal precipitation (Glisan, 2007; Whan et al., 2016). Many of these preferential areas for blocking occurrence tend to represent an extension of the geographical location of enhanced subtropical ridge activity (Sousa et al., 2021). Moreover, Northern Hemisphere blocking is also observed over the Pacific basin – both over the west Pacific and the east Pacific. In comparison to the Atlantic and European counterparts being more common in the period from winter to spring, Pacific blocks are most frequent in spring (Barriopedro et al., 2006). The different blocking areas dealt with in this study are shown in Fig. 1 (Shabbar et al., 2001; Buehler et al., 2011; Luo et al., 2016; Rohrer et al., 2019; Sousa et al., 2021). These are Greenland (GL), the North Atlantic (N-ATL), Europe (EU), Scandinavia (SCAN), the subtropics (SUBTROP) and the Ural Mountains (URAL). The areas shown are partly overlapping. Scandinavian blocking can be understood as a subset of European blocking events, the southern parts of the North Atlantic and European blocking overlap with the area where subtropical high ridges can occur, and the southern part of the Greenland blocking area falls within the North Atlantic area, which means that southern Greenland blocks can also be categorized as North Atlantic blocks. Please note that the precise definitions of these areas vary slightly in their boundaries between different studies (e.g., Rohrer et al., 2019; Wachowicz et al., 2021), which may have an influence on the results.
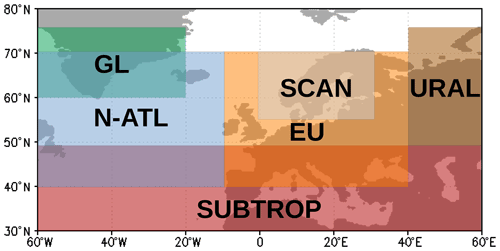
Figure 1Regions over the Euro-Atlantic sector where blocking frequently occurs: Greenland (GL, green box), North Atlantic (N-ATL, blue box), Europe (EU, orange box), Scandinavia (SCAN, beige box) subtropics (SUBTROP, red box) and Ural Mountains (URAL, brown box) (Shabbar et al., 2001; Buehler et al., 2011; Luo et al., 2016; Rohrer et al., 2019; Sousa et al., 2021).
2.2 Relevant mechanisms for blocking formation, maintenance and decay
A variety of mechanisms have been linked with blocking, and the balance of mechanisms differs for blocking systems of different types and regions. The interaction of Rossby waves of different scales is a common feature of many mechanisms (Nakamura et al., 1997), often leading to wave breaking and irreversible deformation of PV contours (Hoskins et al., 1985; Altenhoff et al., 2008). This can include quasi-stationary waves originating in the tropics as well as mid-latitude transients (Hoskins and Sardeshmukh, 1987). Blocking systems often occur in regions of weak or diffluent flow which have a lower capacity for wave propagation (Gabriel and Peters, 2008; Nakamura and Huang, 2018), and these regions are often modulated by stationary waves forced by thermal contrasts and continental elevation (Tung and Lindzen, 1979; Austin, 1980).
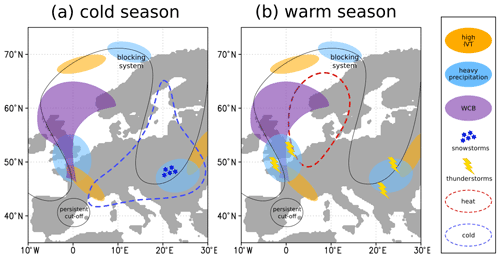
Figure 2Schematic illustration of a blocking system (black line, indicating a geopotential height or PV contour) and some associated surface extremes during (a) the cold season (October–March) and (b) the warm season (April–September). Rossby wave breaking occurs on the flanks of the block, leading to (persistent) cutoff systems in this area. Blue stars show areas where snowstorms are observed (eastern flank of the block). Areas with heavy precipitation are marked in light blue (poleward edge of the ridge and at both flanks). Areas with high integrated water vapor transport (IVT) are illustrated in orange. Thunderstorm activity is marked by yellow lightning bolt symbols. The position of a warm conveyor belt appears in purple. Areas with temperature extremes are marked with dashed lines (red for heat waves and blue for cold spells).
The blocking anticyclone comprises a broad, uniform area of low-PV air which has often been advected poleward in the upper troposphere (Crum and Stevens, 1988). Latent heating can also contribute to the formation of such negative PV anomalies by enhancing the transport of lower tropospheric air upwards along warm conveyor belts and into the upper anticyclone, where it arrives with low PV values (see purple area in Fig. 2) (Madonna et al., 2014; Methven, 2015; Pfahl et al., 2015). This is particularly common in blocking systems forming within or just downstream of the oceanic storm tracks (Steinfeld and Pfahl, 2019). Strong cyclone activity in the region upstream is also known to contribute to blocking formation through adiabatic processes as well (Colucci, 1985).
The low-PV air mass can be supported by exchange processes between the blocking system and transient eddies, i.e., fast-moving short-lived synoptic-scale systems (Berggren et al., 1949). This can involve a complete replacement of the original air mass by a subsequent wave breaking event (Hoskins et al., 1985) or a subtler “drip-feeding” of low-PV air (Shutts, 1983). While the importance of transient eddy feedbacks seems clear, the precise mechanisms supporting this are still debated (Luo et al., 2014; Wang and Kuang, 2019), and the feedbacks maintaining the displaced jet may be important as well as those acting on the blocking anomaly itself (Berckmans et al., 2013).
The mechanisms involved in a blocking system can relate in some cases directly to its impacts. For example, storm activity upstream of the blocking system can lead to high wind and precipitation impacts there (e.g., Lenggenhager and Martius, 2020), while in other cases amplified planetary waves can be associated with simultaneous impacts in remote regions (e.g., Kornhuber et al., 2020).
2.3 Predictability
Blocking is often considered a challenge for prediction systems, but this is only true in some regards. Firstly, blocking can be associated with hemispheric-scale teleconnections, often with influences detected in the tropics (Hoskins and Sardeshmukh, 1987; Moore et al., 2010; Henderson et al., 2016; Gollan and Greatbatch, 2017; Drouard and Woollings, 2018; Parker et al., 2018). At least for these events, the intrinsic predictability of the physical system may be relatively high, although biases in models can hinder the realization of this potential, for example by misrepresenting tropospheric waveguides (O'Reilly et al., 2018; Li et al., 2020).
The representation of blocking by numerical models has been a long-standing concern in both weather forecasting and climate simulation contexts (e.g., Tibaldi and Molteni, 1990; Pelly and Hoskins, 2003b). Considerable improvement has been made as models have developed (Davini and D'Andrea, 2020), partly through improved resolution (Schiemann et al., 2017) but also through improvements to numerical schemes (Martínez-Alvarado et al., 2018). An overview focused on climate models is provided by Woollings et al. (2018). While many models continue to exhibit serious biases in blocking, it is becoming apparent that only over Europe do models systematically underestimate blocking compared to observations (Patterson et al., 2019; Davini and D'Andrea, 2020), highlighting the importance of the northern stationary waves and/or specific local processes.
Recent efforts to archive forecasts of weather prediction systems, or in some cases re-forecasts, have shown that blocking remains a challenge on the medium-range weather timescale. In many cases, the forecast errors are larger for European blocking compared to other regimes, particularly during the transition phases into or out of a blocking regime (Hamill and Kiladis, 2014). Conversely, during the maintenance phase of a blocking system, the errors are often smaller, although the persistence of blocking systems can still be underestimated (Ferranti et al., 2015). Blocking forecast errors remain a concern, but, for perspective, the contrast to other regimes is often subtle and requires a large sample of forecasts for statistical significance (Matsueda and Palmer, 2018). While there is room for further improvement, blocking systems are often successfully predicted, and this can provide early warnings of related extreme weather.
Several recent studies give specific examples of physical processes which can be improved in models to enable better prediction of blocking. These include diabatic processes upstream of blocking systems (Rodwell et al., 2013; Quandt et al., 2019; Maddison et al., 2020), orographic effects (Jung et al., 2012; Berckmans et al., 2013; Pithan et al., 2016) and hemispheric Rossby wave teleconnections, often to tropical structures such as the Madden–Julian Oscillation (Hamill and Kiladis, 2014; Parker et al., 2018).
The frequent connection of blocking to hemispheric, and particularly tropical dynamics, provides an opportunity for skillful predictions of blocking variability on monthly, seasonal and even interannual timescales, which is just starting to be realized (Athanasiadis et al., 2014, 2020). Hence, blocking processes could contribute to skillful predictions of related impacts on these timescales, although such predictions would be inherently probabilistic forecasts of, for example, seasonal risk of heat waves or floods.
2.4 Impact on surface extremes
The strong interest in blocking and its predictability is related to the occurrence of associated high-impact weather (e.g., Matsueda, 2009). To be more precise, blocking is mainly associated with temperature (e.g., Quandt et al., 2017) as well as hydrological extremes (e.g., Lenggenhager et al., 2019). Blocking has also been associated with other extremes such as marine heat waves (e.g., Rodrigues et al., 2019), episodes of low air quality (e.g., Pope et al., 2016; Webber et al., 2017) and with wind extremes to a lesser extent (e.g., Pfahl, 2014).
Using the example of an Omega block, Fig. 2 shows possible associated surface extremes depending on the season. Please note that although these extremes are shown schematically in the same plot, they do not necessarily occur simultaneously, even if this has been observed in some sporadic circumstances (e.g., Russian heat wave and Pakistan floods in summer 2010). During the cold season (from October to March), low-temperature anomalies may be observed in the southern and the eastern parts (at the eastern flank) of the blocking system (Fig. 2a). In addition, there are also cases where snowstorms have been observed at the eastern flank of the blocking system. During the warm season (from April to September), heat waves may develop below the blocking ridge (Fig. 2b). Sometimes these heat waves co-occur with droughts. Moreover, thunderstorms are possible at the eastern and at the western flanks of the blocking system. Heavy rainfall events, which may lead to flooding and which are co-located with areas of high integrated water vapor, are possible at the flanks and near the poleward edge of the blocking ridge during the whole year. The specific location of temperature and precipitation anomalies does, however, depend on the positioning and type of blocking. For example, Sousa et al. (2021) discuss how different phases of a blocking life cycle over western Europe (from an open ridge stage to the posterior stages of Omega and Rex block) during winter impose very distinct regional impacts, a fact the authors explain with the varying morphology of the blocking structure and the corresponding synoptic environment.
As shown in the Fig. 2 and as reviewed below, the impacts of blocking can vary considerably between seasons and regions, but many impacts arise from one characteristic: the persistence of blocking systems. This persistence is a hallmark of blocking and arises from the dynamics of low-frequency waves, irreversible wave breaking and eddy feedbacks (Hoskins et al., 1985; Pelly and Hoskins, 2003a; Nakamura and Huang, 2018; Drouard et al., 2021). Blocking persistence can lead to extended periods of extreme weather and so has a clear societal impact. While the severity of the meteorological impact can be related to the number of blocking days (Schaller et al., 2018; Lenggenhager et al., 2019), it is not clear that the persistence of individual blocking events is key here (Chan et al., 2019) or whether the recurrence of blocking may have similar impacts (Woollings et al., 2018). Moreover, the stalling of cyclones upstream of a blocking system as observed, for example, in winter 2013–2014 over Great Britain (Priestley et al., 2017) is a process which does not necessarily require the blocking system to be persistent.
To give indicative numbers, an average blocking system might last 7–10 d and the most extreme events for 2–3 weeks, though there are significant differences between the statistics of different metrics. Also Drouard and Woollings (2018) argued that recurrence of blocking was more important than persistence per se in some cases, such as the Russian heat wave of 2010. They suggested that a seasonal count of the occurrence of blocking was a useful metric for quantifying such impacts.
3.1 Overview
Heat waves and cold spells are respectively long-lasting periods of unusually high or low temperatures (e.g., Robinson, 2001). The extremely high temperatures during heat waves lead to heat stress and thus to a reduction in human comfort (e.g., Koppe et al., 2004; Robine et al., 2008; Perkins, 2015). In addition, they increase the risk of heat-related illnesses and mortality (e.g., Gasparrini et al., 2015). Also cold spells can cause substantial health risks (e.g., Charlton-Perez et al., 2019). Based on data from 13 countries, it was found that 7 % of the total mortality between 1985 and 2002 was due to extreme coldness (Gasparrini et al., 2015). Moreover, cold spells also influence everyday life by affecting power supply or public transport. Both heat waves and cold spells often occur in parallel with droughts, which are periods with little to no precipitation often leading to additional and amplified impacts (see Sect. 4) (e.g., Schumacher et al., 2019; Sousa et al., 2020).
Heat waves in Europe are typically co-located with high-pressure anomalies and thus associated with anticyclonic circulation throughout the troposphere (Meehl and Tebaldi, 2004; Cassou et al., 2005; Stefanon et al., 2012; Tomczyk and Bednorz, 2016; Zschenderlein et al., 2019). Accordingly, blocking, which is characterized by persistent anticyclonic flow anomalies, strongly correlates with the occurrence of European temperature extremes in summer (Fig. 2b). More than 50 % of the most extreme (above the 99th percentile) 6-hourly maximum temperature events in many regions in central and eastern Europe and more than 80 % in parts of Scandinavia and Scotland have been shown to co-occur with blocking (defined in terms of quasi-stationary PV anomalies) (Pfahl and Wernli, 2012). In southern Europe, heat waves typically occur in association with extended subtropical ridges (Sousa et al., 2018), which often do not lead to the overturning of geopotential contours and flow reversal that characterizes classical blocking patterns but may still be linked to persistent PV anomalies further north (Pfahl, 2014). Similar to other properties of blocking, the association with heat waves thus depends on the blocking index: anomaly-based indices tend to show stronger correlations with heat waves than blocking indices solely based on flow reversal or wave breaking (Chan et al., 2019).
European cold spells are associated with mid- and high-latitude blocking over the North Atlantic as well as over the European continent. However, in the most cases, the cold anomaly is not located directly below the blocking anticyclone but downstream or south of it (Fig. 2a). Over the North Atlantic, blocking is strongly correlated with the negative phase of the NAO that itself is associated with the development of European cold spells. The synoptic pattern during NAO− provides diffluent flow conditions which are favorable for the onset and maintenance of blocking systems (Luo et al., 2015). However, it is generally difficult to consider the North Atlantic blocking and NAO− separately, as the flow configuration during NAO− itself can be defined as a blocking pattern (e.g., Woollings et al., 2008). This results in the development of negative NAO index values during North Atlantic blocking episodes (Croci-Maspoli et al., 2007). The frequency of winter cold anomalies over Europe depends on the exact location of the blocking system (Sillmann et al., 2011; Brunner et al., 2018): the frequency is increased across most of Europe for blocking over Greenland, while the influence is largest over central Europe for blocking over the North Atlantic (the influence is larger for systems closer to the continent) and Scandinavia. However, the same blocking system may favor cold anomalies at different locations across Europe (Pfahl, 2014). In numbers, up to 70 % of winter cold spells in central Europe can be associated with a blocking system anywhere between 60∘ W and 30∘ E (Brunner et al., 2018).
3.2 Dynamics
European heat waves are created by two main processes: heat accumulation due to atmospheric transport and diabatic heating via radiation and surface fluxes (Miralles et al., 2014). Blocking can be conductive to both of these processes, which explains its strong connection to heat waves (Pfahl and Wernli, 2012; Sousa et al., 2018). Although blocking formation itself is often connected to the northward advection of subtropical air masses in the middle and upper troposphere (Nakamura et al., 1997), recent Lagrangian studies (Bieli et al., 2015; Santos et al., 2015; Zschenderlein et al., 2019) have shown that horizontal advection from lower latitudes is only of secondary importance for the near-surface air under heat wave conditions. Rather, the accumulation of heat near the surface is due to descent and adiabatic warming within the blocking anticyclones or subtropical ridges. In addition, this descent is also related to clear-sky conditions that favor surface heating by solar radiation during daytime, counteractive cooling during nighttime and diabatic heating of the near-surface air through amplified sensible heat fluxes. This diabatic heating can be further enhanced by a feedback mechanism with soil moisture (Fischer et al., 2007; Miralles et al., 2019): given the lack of precipitation in the blocking region (see also Sect. 4), soil moisture is depleted and a larger fraction of the surface-atmosphere heat flux occurs in the form of sensible (in contrast to latent) heat. Soil–moisture feedback and atmospheric heat transport can also act in concert when sensible heat is advected towards heat wave areas from upstream regions affected by drought (Schumacher et al., 2019) and potentially also through feedbacks of the altered surface fluxes on the atmospheric circulation (Merrifield et al., 2019). The physical mechanisms through which blocking influences heat waves can be amplified due to the persistence of blocking. The lifetime of heat waves increases when they co-occur with a blocking system (Röthlisberger and Martius, 2019), favoring the long-term accumulation of heat.
Cold spells can be favored downstream of a blocking system by the horizontal advection of cold air from higher latitudes or cold land masses (Arctic and Russia) (Bieli et al., 2015; Santos et al., 2015; Demirtaş, 2017; Sousa et al., 2018). When the cold air (originating, e.g., in the Arctic region) is transported to the target area, it typically descends, leading to a warming of the air masses due to adiabatic compression and turbulent mixing with warmer air (Bieli et al., 2015). In addition, blocking systems occurring over the northern North Atlantic can trigger the equatorward displacement of the North Atlantic storm track. The shift of the storm track results in a more southward passage of cyclones towards Europe (Pfahl, 2014). Since cyclones moving over Europe can favor the advection of cold continental air masses from northeastern and eastern areas behind their cold fronts, the exact cyclone track has an influence on where a cold spell will potentially develop. Furthermore, the development of cold anomalies in winter can be modulated by persistent clear-sky conditions associated with a blocking anticyclone (Trigo et al., 2004; Demirtaş, 2017). The cloudless sky leads to a strong cooling due to outgoing longwave radiation during nights (diabatic cooling). This process is relevant directly below the blocking anticyclone; thus, it is an in situ process. However, there is a temperature increase associated with adiabatic heating due to subsidence in the area of the blocking anticyclone, which may counteract the diabatic cooling (Sousa et al., 2018). Comparing these mechanisms to each other, it was found that the advection of cold air from north and northeast is most important for the evolution of European cold spells (Trigo et al., 2004; Pfahl, 2014; Bieli et al., 2015; Sousa et al., 2018). Cold spells need some time to evolve during blocking situations (Buehler et al., 2011), making the development of a cold anomaly more probable during long-lasting blocking events.
As blocking anticyclones are typically embedded in larger-scale Rossby waves, the relationship between temperature extremes and blocking also translates into a linkage of heat and cold spells to Rossby wave activity. European heat extremes often occur in summer during periods of regionally enhanced Rossby wave activity over the Eurasian continent, while winter cold spells in western Europe and parts of the Mediterranean are more associated with enhanced Rossby wave activity over the North Atlantic (Röthlisberger et al., 2016; Fragkoulidis et al., 2018). The persistence of summer hot spells (winter cold spells) can be increased (decreased) due to recurrent Rossby wave patterns that amplify in the same geographical region (Röthlisberger et al., 2019). Quasi-resonance of hemispheric wave activity (Petoukhov et al., 2013) may lead in summer to simultaneous heat waves in several regions (Kornhuber et al., 2020). Finally, as for other blocking systems (see Sect. 2), the dynamics of anticyclones associated with European summer heat waves can be affected by latent heat release in ascending air masses embedded in upstream wave packets (Zschenderlein et al., 2020).
3.3 Case studies
The cases discussed in this section, Sects. 4.3 and 5, are examples for surface extreme events that were triggered or at least influenced by blocking (see Tables 1, 2 and 3). Different approaches were chosen in the studies cited to show this connection: on the one hand, methods such as the calculation of backward trajectories, clustering or correlation analyses were used. On the other hand, there are some studies on surface extreme events in which a synoptic analysis was made, showing that blocking dominated the flow pattern, from which it was assumed that the extreme event was influenced accordingly.
Green (1977)Ellis et al. (1980)De Bono et al. (2004)Miralles et al. (2014)Kron et al. (2019)Barriopedro et al. (2011)Grumm (2011)Lassnig et al. (2014)Lhotka and Kysely (2015)Bastos et al. (2020)Spensberger et al. (2020)Lejenäs (1989)Cattiaux et al. (2010)Seager et al. (2010)Wang et al. (2010)DWD (2012)de Vries et al. (2013)Planchon et al. (2015)Anagnostopoulou et al. (2017)Karpechko et al. (2018)Ferranti et al. (2019)Table 1Examples for temperature extreme events in Europe associated with blocking. The abbreviations for the blocking region refer to Fig. 1. Losses are not inflation-adjusted. Please note that the information in the column Damage is partly not complete, as not all information is available in the corresponding literature.
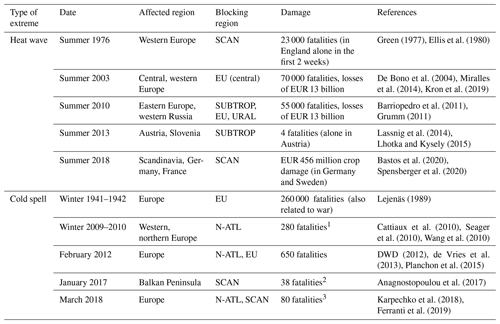
1 https://www.spiegel.de/panorama/katastrophenstudie-die-liste-der-extremwinter-a-812855.html (last access: 19 November 2021).
2 https://www.n-tv.de/panorama/38-Menschen-erfrieren-in-Europa-article19507451.html (last access: 19 November 2021).
3 https://www.munichre.com/topics-online/de/climate-change-and-natural-disasters/natural-disasters/natural-catastrophes-first-half-of-2018.html (last access: 19 November 2021).
Table 2As in Table 1 but for hydrological extremes.
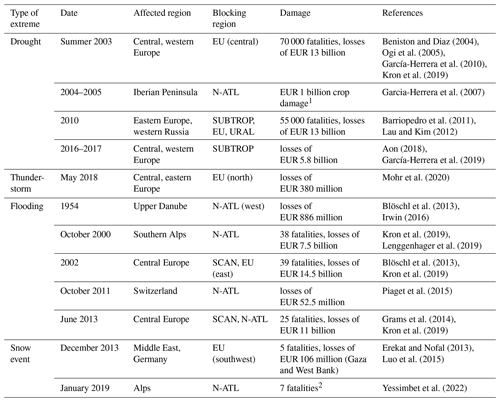
1 https://www.n-tv.de/panorama/Iberische-Halbinsel-trocknet-aus-article149751.html (last access: 20 November 2021),
2 https://www.bbc.com/news/world-europe-46780856 (last access: 20 November 2021).
3.3.1 Heat waves
We briefly discuss several historical case studies to highlight the case-to-case variability in the dynamical processes leading to European heat waves and the role of blocking. The most prominent and most severe European heat waves occurred in summer 2003 in western and central Europe (e.g., Black et al., 2004; Fink et al., 2004; Schär et al., 2004) and in summer 2010 in eastern Europe (Fig. 3a) (e.g., Barriopedro et al., 2011; Grumm, 2011). In 2003, record-high temperatures were measured in June and August, which were dominated by anticyclonic weather regimes (Fink et al., 2004). While the monthly-mean circulation in June was characterized by a broad ridge centered over central Europe, blocking was dominant particularly for the first half of August (Black et al., 2004). Warm air advection may have played a role for the earlier phases of the heat wave in June (Fink et al., 2004), but during August the flow over France (in the center of the blocking system) was dominated by stagnant air masses recirculating and descending within the blocking anticyclone (Black et al., 2004). Positive anomalies in outgoing longwave and incoming shortwave radiation associated with clear-sky conditions point to an important role of radiative forcing, and precipitation deficits started already in April, leading to a positive soil–moisture feedback that strongly amplified the heat wave (Black et al., 2004; Fink et al., 2004; Fischer et al., 2007; Miralles et al., 2014).
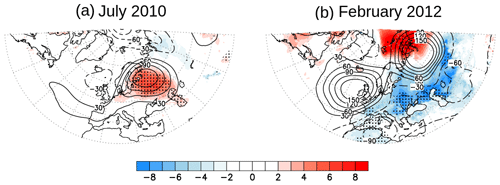
Figure 3Monthly 2 m temperature (in K, shading) and 500 hPa geopotential height anomalies (in m, contours) based on ERA5 data (Hersbach et al., 2020) (a) in July 2010 (in association with the 2010 heat wave) and (b) in February 2012 (in association with the 2012 cold spell) are shown. Dots mark areas exceeding the 2σ level of the 2 m temperature climatology (1991–2010).
The 2010 heat wave mainly affected eastern Europe and western Russia and was associated with a strong anticyclonic circulation anomaly (e.g., Grumm, 2011) (see also Fig. 3a) and a reversal of the meridional geopotential height gradient at 500 hPa characteristic for blocking during most of the period between late June and early August 2010 (Lau and Kim, 2012; Schneidereit et al., 2012). Moreover, the event has been characterized by a clear positive anomaly in the frequency of subtropical ridges and Omega-type blocks in this longitudinal sector (Sousa et al., 2021). This blocking anomaly was unprecedented in particular in the eastern part of the region around 50∘ E. In addition, it was linked to a quasi-stationary Rossby wave train over the Euro-Atlantic sector and, more generally, over the Northern Hemisphere, consistent with a La Niña teleconnection (Trenberth and Fasullo, 2012; Drouard and Woollings, 2018). The desiccating soils and enhanced surface sensible heat fluxes played an important role (Lau and Kim, 2012; Miralles et al., 2014; Hauser et al., 2016).
In addition to these most prominent cases, blocking and extended ridges also played a role for other European heat waves (see Table 1). For example, the heat wave in July 1976 affecting western Europe occurred during blocked conditions (Green, 1977), while a heat wave over central Europe in 2013 was associated with a subtropical ridge extending northeastward from the western Mediterranean (Lhotka and Kysely, 2015). The late-summer heat wave in 2016 was mainly driven by subsidence and diabatic heating in the boundary layer within positive geopotential anomalies that were embedded in eastward-propagating Rossby wave packets (Zschenderlein et al., 2018). On the other hand, Scandinavian blocking was associated with the 2018 heat waves over Scandinavia, northern Germany and France (Spensberger et al., 2020). These cases also illustrate that the location of the high-pressure anomaly largely determines which region is affected by a heat wave (see Fig. 2b).
Marine heat waves can also be related to blocking activity. Regarding the 2003 case, an impact on the sea surface temperatures (SSTs) of the Mediterranean Sea is documented (Sparnocchia et al., 2006; Olita et al., 2007). The large-scale blocking over France in 2003 increased air temperature and reduced wind speed (leading to a reduction of all components of the upward heat flux), which were ultimately responsible for the abnormal positive SST anomalies over the Mediterranean Sea. Another example of the importance of blocking activity is the 2012 northwest Atlantic marine heat wave (K. Chen et al., 2015), when persistent atmospheric ridges and blocking through the winter reduced wintertime heat loss from the ocean to the atmosphere (Holbrook et al., 2020).
3.3.2 Cold spells
In February 2012, large parts of Europe were affected by extremely low surface temperatures (in many regions 10 ∘C below average, Fig. 3b) accompanied by heavy snowfall (de Vries et al., 2013; Demirtaş, 2017). Even Italy experienced minimum temperatures of −15 ∘C (WMO, 2015). The cold period affected the traffic sector, health and agriculture (e.g., Planchon et al., 2015). The occurrence of cold anomalies across Europe was triggered by a persistent ridge–trough–ridge pattern (Demirtaş, 2017). Both ridges were blocking systems, one amplified northeast–southwest tilted ridge over the Atlantic and one Omega blocking high over Siberia (see Fig. 3b). These upstream and downstream ridges favored the persistence of the trough in between (Demirtaş, 2017) and, thus, the continuous advection of cold air from northern regions. In 2017, the synoptic pattern over Europe was similar to 2012, with an extension of the Siberian anticyclone towards Scandinavia that blocked the zonal flow and triggered the advection of cold air from the north (Anagnostopoulou et al., 2017). Compared to 2012, the air masses in January 2017 arrived from much higher latitudes (Anagnostopoulou et al., 2017) and favored the evolution of a cold episode over the Balkan Peninsula which was extreme both due to its magnitude and long duration (Anagnostopoulou et al., 2017).
On the other hand, the European cold spell in March 2018 was primarily triggered by the negative phase of the NAO, which was probably preconditioned by a sudden stratospheric warming event in mid-February (Karpechko et al., 2018). Although the negative NAO was the dominating flow feature in that case, the extension of the cold spell was also influenced by Scandinavian blocking. At the end of February, shortly before the shift of the NAO from its positive to its negative phase, a blocking system over Scandinavia evolved, advecting the polar air southwestward (Ferranti et al., 2019; Kautz et al., 2020).
Three cold outbreaks in western and northern Europe between late December 2009 and mid-January 2010 were also associated with an extremely persistent negative NAO phase (Cattiaux et al., 2010; Seager et al., 2010; Wang et al., 2010; Santos et al., 2013). The negative NAO favored northerly surface wind anomalies leading to a southward advection of cold Arctic air (Wang et al., 2010). The low-temperature anomalies coincided with precipitation deficits but an unusual persistence of snow cover (Seager et al., 2010). In addition, this winter has the second-highest frequency of North Atlantic blocking since 1949, which is related to the negative NAO phase, as it generally favors for the development of blocking over the North Atlantic (Cattiaux et al., 2010). The low temperatures of this winter were mainly connected to NAO− and less to, for example, Scandinavian blocking (Cattiaux et al., 2010), which was relevant in the 2018 cold spell. However, no study investigates the role of certain blocking systems for the 2009–2010 winter in detail.
In winter 1941–1942 stationary troughs over Europe brought low temperatures and shifted storms tracks affecting the war (Lejenäs, 1989). Another example for an extreme cold event associated with the occurrence of a blocking system and a blizzard was observed in March 1987 (Tayanc et al., 1998). Since this event was accompanied by heavy snowfall, it is described in Sect. 4.
3.4 Challenges
The development of temperature extremes depends strongly on the persistence and location of blocking. Longer and quasi-stationary blocking periods provide long-lasting favorable conditions for the occurrence of cold spells/heat waves. While the relationship between blocking and temperature extremes is often given, there is a high case-to-case variability both in the phasing and other influences (e.g., soil moisture). Measurement campaigns or sensitivity experiments with numerical models could help to further investigate the multiple interactions. The main challenge here is to cover all relevant components and process chains across a multitude of spatial scales and timescales.
4.1 Overview
In an early blocking study, Rex (1950) described the effects of six cases of blocking on the sub-seasonal precipitation distribution over Europe. Conditions were anomalously dry underneath the blocking anticyclones and anomalously wet to the west and to the east of the blocking anticyclones (see Fig. 2). Rex attributed these precipitation anomalies to the blocking modulating the location of storm tracks in the blocked longitudes. Namias (1964) and Trigo et al. (2004) confirmed the strong link between blocking occurrence over Europe and precipitation anomalies using multi-annual data sets. A strong dependence of the location of precipitation anomalies over Europe on blocked longitude exists (Yao and De-Hai, 2014; Sousa et al., 2017). How the link between blocking and precipitation anomalies translates to hydrological extremes such as heavy precipitation, droughts and floods is discussed in this section.
Droughts have a negative influence on water quantity and quality, thus affecting diverse socio-economic activities and ecosystems. For example, water deficits can lead to crop failure with devastating effects for agriculture (Masih et al., 2014) and negatively influence power generation (Pfister et al., 2006). Dry summerly conditions may also be favorable for wildfires (Haines, 1989) and other massive air pollution events, with strong impacts on human health (Finlay et al., 2012; Péré et al., 2014; Athanasopoulou et al., 2014). Furthermore, dry spells lead to enhanced soil–atmosphere feedback processes and thus to amplified heat waves (see Sect. 3) (Miralles et al., 2014; Schumacher et al., 2019). In this article, drought refers to meteorological drought, where the atmospheric conditions result in the absence or decrease in precipitation, which in the long run can result in an agricultural drought and/or hydrological drought (Heim, 2002).
Europe experiences diverse impacts from blocking in terms of drought occurrence. Mid- and high-latitude blocking systems have been shown to severely reduce precipitation in the regions directly below the high-pressure system (Sousa et al., 2017). Also, the role of low-latitude blocking and/or subtropical ridges has been discussed, showing that these lower-latitude high-pressure systems are the main drivers of water scarcity in southern Europe (Santos et al., 2009; Sousa et al., 2017). The impact of blocking and ridge episodes in terms of water availability and drought intensity varies with their season of occurrence. For example, the more even distribution of seasonal rainfall in central and northern Europe leads to similar impacts of blocking in different seasons; thus, severe drought occurrence is dependent on very prolonged large-scale anomalies imposed by blocking systems. However, many European regions' water availability relies on more concentrated precipitation seasons, thus being more susceptible to drought in the case of shorter blocking events coinciding with their precipitation season. This is notable, for example, in the Iberian Peninsula, where annual rainfall totals are highly dependent on extended winter rainfall (October to March), or in eastern Europe, where more substantial summer rainfall constitutes a significant part of annual totals.
Floods are one of the most disastrous weather-related hazards in central Europe (e.g., Alfieri et al., 2018). Flood events and heavy precipitation (including extreme snowfall) can result in casualties; in high economic losses; and in substantial damages to housing, infrastructure and transport. Long-lasting precipitation periods, serial clustering of heavy-precipitation events or very intensive (convective) rainfall events can all trigger floods (e.g., Merz and Bloschl, 2003; Froidevaux et al., 2015). In addition to precipitation, soil moisture content and snowmelt may play important roles as hydrological precursors to floods (e.g., Merz and Bloschl, 2003). Blocking can influence all of these factors. However, the focus of this section is on the link between blocking, heavy precipitation, extreme snowfall and floods.
Blocking affects regional-scale heavy precipitation in Europe (e.g., Lenggenhager and Martius, 2019). Blocking systems change the odds of regional-scale 1, 3 and 5 d heavy precipitation both in the summer season and the winter season. The odds of heavy-precipitation events are reduced in the blocked area and high in the areas southwest to southeast of the blocking anticyclone and sometimes along the northern edge of the blocking anticyclone (see also Fig. 2). Often areas of increased odds of heavy precipitation coincide with the location of the midlatitude cyclone track and hence the passage of fronts and warm conveyor belts, hinting at the important role of storm track modulation by the blocking systems (Sousa et al., 2017; Lenggenhager and Martius, 2019). This is particularly relevant for southern Europe (including the Mediterranean area), where classical blocking configurations can lead to above-average rainfall and extreme precipitation events.
4.2 Dynamics
The quasi-stationary nature of blocking imposes persistent large-scale circulation anomalies, dominated by a large area of subsidence and a stable atmosphere in the center of the blocking system. At the same time, surface cyclones are guided along the edges of the blocking systems resulting in active storm tracks both to the north and the south of a blocking. This bifurcation of the storm tracks associated with blocking has been identified as the most general dynamical pattern linking blocking and (heavy) precipitation over Europe (Rex, 1950; Sousa et al., 2017; Lenggenhager and Martius, 2019). Blocking systems affect the stationarity and pathways of cyclones in their surroundings (Berggren et al., 1949; Nakamura and Wallace, 1989; Swanson, 2002; Booth et al., 2017; Sousa et al., 2017; Nakamura and Huang, 2018; Lenggenhager and Martius, 2019). In addition, blocking circulation affects atmospheric moisture transport and thereby heavy precipitation (e.g., Piaget et al., 2015; Sousa et al., 2017; Lenggenhager and Martius, 2019). For example Piaget et al. (2015) identified an atmospheric river steered towards Switzerland along the northern edge of a blocking anticyclone as an important driver for a local flood event, and Lenggenhager and Martius (2019) identified high moisture transport along the northern edge of a blocking anticyclone to be linked to heavy precipitation along the west coast of Scandinavia (see also Fig. 2).
The lack of cyclones and the prevailing subsidence occurring in the large area under the blocking center leads to reduced (or even virtually suppressed) precipitation (Yao and De-Hai, 2014; Sousa et al., 2017). In this sense, large-scale downward motion is the primary atmospheric mechanism leading to surface water shortages (and eventually droughts) during persistent blocking episodes.
For a more detailed process discussion we first focus on drought conditions. A strong zonal circulation associated with a positive NAO phase, which reflects a more stable stratospheric polar vortex, inhibits the occurrence of significant Rossby wave breaking (RWB) episodes, thus leading to less favorable conditions for blocking episodes (Masato et al., 2012) and, consequently, to fewer drought-prone conditions in most areas of Europe. However, low-latitude structures (in particular subtropical ridges) are frequently the initial stage of an RWB event, as they remain connected to the subtropical high-pressure belt. This is both the case prior to the occurrence of a cutoff high-pressure system and a subsequent transition to a mature blocking system. During strong zonal flows, incipient RWB might occur, but this generally does not lead to mature and persisting blocking systems. These initial phases of a blocking life cycle are also important contributors for persistent stable and dry episodes, in particular in southwestern Europe, as they tend to block Atlantic frontal activity from reaching the region (Santos et al., 2009; Sousa et al., 2017). Under these configurations, a more constrained jet stream can be found upstream of the ridge, leading to a significant wet/dry north/south dipole in terms of precipitation anomalies. Under less intense zonal flows, conditions are more favorable for full RWB episodes to develop over the Euro-Atlantic sector, thus leading to more frequent mid- and high-latitude blocking episodes (Rex type) and thus reverting the aforementioned precipitation anomaly dipole. However, it has been shown that Omega blocks, which present a subtropical connection (more similar to amplified ridges), tend to produce higher rainfall deficits in southern Europe, when compared to Rex blocks, where a more intense southerly branch of the split jet stream can be observed (Sousa et al., 2021).
While these dynamical features can be observed throughout the year, seasonal intricacies exist. For example, cold advection near the eastern flank of a blocking system during winter might lead to widespread negative surface temperature anomalies over the European continent increasing vertical stability (Sillmann et al., 2011; Sousa et al., 2018). Also during the warmer months, positive feedbacks in terms of surface drying can be observed as a consequence of blocking (Fischer et al., 2007; Seneviratne et al., 2010). Strong radiative fluxes under clear-sky conditions imposed by the blocking structure lead to reduced rainfall, and soil moisture (enhanced evapotranspiration) can lead to severe and detrimental soil desiccation processes. Previous works have shown that a surface-initiated process like this can be amplified and propagate upwards, also amplifying pressure anomalies and consequently intensifying a warm core anticyclone (Miralles et al., 2014; Schumacher et al., 2019).
We now evaluate the links between blocking and heavy precipitation and flooding. Blocking influences the location of cyclones and thereby the positions of warm conveyor belts (Grams et al., 2014), fronts and atmospheric rivers (Pasquier et al., 2019), which are all important weather features that directly force precipitation (e.g., Pfahl and Wernli, 2012). The detailed mechanisms leading to blocking-related heavy precipitation differ regionally and include important interactions with the local topography. However, there is a general pattern of instability which is a key ingredient for blocking-related precipitation in southern Europe, with moisture availability being another key ingredient for blocking-related precipitation in northern Europe (Sousa et al., 2017).
Links between heavy precipitation and blocking are present across Europe. A high frequency of blocking over Scandinavia results in a high frequency of extreme summer precipitation in Romania and the eastern Mediterranean (Rimbu et al., 2016) but also in a significant increase in the odds of regional-scale heavy precipitation over Greece and the central Mediterranean (Lenggenhager and Martius, 2019). Extreme precipitation over Romania is associated with RWB forming downstream of the blocking anticyclones (Rimbu et al., 2016). Blocking over Scandinavia reduces the odds of heavy precipitation over central Europe, while blocking over the central and eastern Atlantic increases the odds of 1 d heavy precipitation in summer over several regions in Europe (Lenggenhager and Martius, 2019). This increase is partly linked to a modulation of cyclone tracks by these blocking systems.
Blocking also affects the frequency of thunderstorms over Europe during summer (see Fig. 2b) (Mohr et al., 2019) and thereby potentially the distribution and frequency of intense convective precipitation (Mohr et al., 2020) and flash floods. Depending on their location, blocking systems may both increase or reduce the odds of thunderstorms (Mohr et al., 2019). Blocking over the eastern North Atlantic suppresses the thunderstorm activity over central Europe due to northerly advection of colder and more stable air masses into Europe and subsidence. Blocking systems over the Baltic Sea increase the odds of thunderstorms over western and central Europe by supporting the advection of warm moist and unstable air masses (Mohr et al., 2019).
Blocking may also play an important role in flood occurrence, particularly in the case of intense and/or long-lasting precipitation. A detailed analysis of the large-scale weather situation during 24 major flood events in Switzerland between 1868 and 2005 revealed that a blocking system was present over Russia for all analyzed summer cases (Stucki et al., 2012). In the flood events where an upper-level cutoff was key to triggering heavy precipitation, the eastward propagation of cutoffs was slowed down by blocking systems (Stucki et al., 2012). A detailed analysis of two severe flood cases in Switzerland in October 1868 (Stucki et al., 2018) and in October 2000 (Lenggenhager et al., 2019) confirms the important role of blocking located east of Switzerland for slowing down the eastward propagation of the upper-level PV streamers and cutoffs that were primarily responsible for the formation of the heavy precipitation. The stalling of these upper-level structures resulted in very high precipitation accumulations and subsequent flooding. An analogous case was the winter 2013–2014 in the UK (see Sect. 5).
Blocking-driven high seasonal precipitation accumulations and heavy precipitation in the cold season may translate to substantial snow accumulations if temperatures are cold enough. Blocking contributes to cold temperatures via the advection of cold air along their eastern flank (see Sect. 3). A distinction must be made between extreme snowfall on seasonal timescales and extreme short-duration snowfall events, both of which can be related to blocking. High snow accumulations during entire winters have been linked to anomalous seasonal blocking frequencies. In the winters of 1958 to 1960 several blocking episodes over Europe resulted in negative snow anomalies in parts of Scandinavia (Namias, 1964). The large snow accumulations in central Europe in winter 2005–2006 were partly related to blocking (Pinto et al., 2007; Croci-Maspoli and Davies, 2009). The Swiss seasonal snow cover is strongly related to Scandinavian blocking (Scherrer and Appenzeller, 2006). Garcia-Herrera and Barriopedro (2006) report a two-way interaction between seasonal Northern Hemisphere snow cover and blocking. First, winter blocking over the Atlantic correlates with spring and summer Eurasian and North American snow cover; second, spring and summer Eurasian and North American snow cover is associated with an anomalous winter Atlantic blocking activity (Garcia-Herrera and Barriopedro, 2006). The underlying processes leading to large precipitation are similar in winter and summer, but for winter blocking the partition between liquid and solid precipitation is influenced by temperature (see Sect. 3). High seasonal accumulations of snow can result in avalanches (Pinto et al., 2007) and may contribute to flooding in the following spring (Stucki et al., 2012).
4.3 Case studies
A list of selected case studies of hydrological extreme events that were caused or influenced by blocking is provided in Table 2, including information on the impacts.
4.3.1 Droughts
Two prominent examples for droughts associated with the occurrence of blocking were observed in 2003 (e.g., Beniston and Diaz, 2004; Cassou et al., 2005; Ogi et al., 2005; García-Herrera et al., 2010) and 2010 (e.g., Barriopedro et al., 2011; Lau and Kim, 2012; Schneidereit et al., 2012). Since these examples are related to mega heat waves, these cases have been already discussed in Sect. 3.
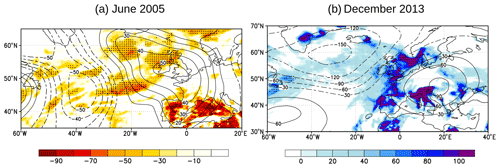
Figure 4Monthly precipitation (in % of the long-term monthly mean, shading) and 500 hPa geopotential height anomalies (in m, contours) based on ERA5 data (Hersbach et al., 2020) (a) in June 2005 (in association with an Iberian drought) and (b) in December 2013 (in association with the 2013 snowstorm in the Middle East) are shown. Dots mark areas exceeding (a) the 1σ or (b) the 2σ level of the precipitation climatology (1991–2010).
We thus highlight other blocking-related droughts in the Mediterranean, a region where climate projections consistently indicate an increased frequency and severity of droughts (Tramblay et al., 2020). One of the most exceptional droughts in the Iberian Peninsula occurred between October 2004 and June 2005 (Fig. 4a). The southern half of Iberia received roughly 40 % of the usual precipitation by June 2005. This was the driest event of the last 140 years, producing major socio-economic impacts particularly due to the large decrease in hydroelectricity and agricultural production in both Portugal and Spain (Garcia-Herrera et al., 2007). While the blocking activity within the North Atlantic sector from October to December was similar to the long-term average (1958–2005), it was exceptional between January and March, generally the wettest period for the western Iberian Peninsula. This was particularly true between 40 and 20∘ W, where its frequency surpassed the 95th percentile of the climatology. In fact, March was characterized by record-breaking number of blocking days, followed by average activity throughout spring (Garcia-Herrera et al., 2007).
A record-breaking drought affected western and central Europe between July 2016 and June 2017, when drought conditions affected over 90 % of central and western Europe, hitting record-breaking values (with respect to 1979–2017) in 25 % of the area with large socio-economic impacts on water supplies, agriculture, and hydroelectric power production and leading to devastating forest fires in Portugal (García-Herrera et al., 2019). This dry period was associated with the occurrence of blocking systems and subtropical ridges which evolved sometimes outside of their typical location (García-Herrera et al., 2019). Moreover, the occurrence of a mega heat wave in June 2017 was associated with a long-lasting subtropical ridge which occurred very early in the season (Sánchez-Benítez et al., 2018).
4.3.2 Heavy precipitation, flood and extreme snowfall
To illustrate the complex interactions between blocking and flood events, several flood case studies are discussed in more detail. We start with a thunderstorm and ensuing flash flood case study. A high number of thunderstorms affected western and central Europe in May 2018, resulting in multiple flash floods (Mohr et al., 2020). A blocking system was located over northern Europe, which affected the thunderstorm in two ways: first, moist, warm, and unstable air masses were advected into western and central Europe along the western flank of the blocking system (see Fig. 2b). Second, high-PV cutoffs repeatedly formed south of the blocking system, locally changing the static stability and thus providing the ideal mesoscale environment for the formation of thunderstorms (Mohr et al., 2020).
We now move on to a rain-on-snow flood case study. The event happened in October 2011 in Switzerland. It was associated with strong northerly flow underneath a trough and the subsequent arrival of an atmospheric river that resulted in substantial flooding (Piaget et al., 2015). A blocking system over the Atlantic was pivotal both for the amplification of a downstream trough and the transport of high amounts of moisture over the Atlantic (Piaget et al., 2015).
The major floods in central Europe in June 2013 were associated with high blocking frequencies over Scandinavia and over the central Atlantic in May 2013 prior to the heavy-precipitation event that triggered the floods (Blöschl et al., 2013; Grams et al., 2014). This flow configuration led to cool and wet conditions over Europe, which led to wet soils prior to the floods (Kelemen et al., 2016). The decisive heavy-precipitation episodes were caused by cyclonic RWB on the upstream flank of the Scandinavian blocking and associated equatorward ascending warm conveyor belts (Grams et al., 2014). Similar large-scale flow situations were associated with three other major flood events (2002, 1954, 1899) in the Upper Danube basin (Blöschl et al., 2013). In 2002, blocking over Scandinavia and eastern Europe slowed the eastward propagation of a cutoff cyclone, resulting in sustained precipitation. In 1954, a blocking system over the western Atlantic and a cutoff low at its downstream flank were pivotal for the floods.
Blocking played a key role in the formation of a flood event in southern Switzerland and northern Italy in October 2000 (Lenggenhager et al., 2019). The flood event resulted from a series of three heavy-precipitation events over the course of a month, with the last event being the most intense and persistent. All heavy-precipitation events were associated with upper-level high-PV streamers and cutoffs that formed downstream of blocking systems over the Atlantic. The last and most persistent heavy-precipitation event was associated with an upper-level cutoff that remained stationary for several days (Pinto et al., 2013). The cutoff's downstream progression was prevented by a downstream blocking system (Lenggenhager et al., 2019). In October 2000 a feedback between heavy-precipitation events and blocking could be identified. A large fraction of the diabatically altered low-PV air that reached and strengthened the blocking systems over the Atlantic and Europe was heated in heavy-precipitation areas (Lenggenhager et al., 2019).
Finally we discuss high-impact snow events. In March 1987 a blizzard affected the eastern Mediterranean and the Balkan region (Tayanc et al., 1998), associated with an intense Mediterranean cyclone which had formed at the eastern flank of a blocking anticyclone over Europe. This cyclone remained quasi-stationary, and its combination with a cold air outbreak along the eastern flank of the blocking system (see Fig. 2a) resulted in high-impact snow accumulations (Tayanc et al., 1998). Similarly, a snowstorm in the Middle East in December 2013 has been linked to Omega-type blocking over Europe, with strong anticyclonic wave breaking along the downstream flank of the blocking system (Fig. 4b) (Luo et al., 2015). The anticyclonically tilted trough supported the snowstorm both through cold air advection and through forced lifting (Luo et al., 2015). Record-breaking snowfall also happened in the northern part of the Alps in January 2019. The snow event was associated with North Atlantic blocking. A persistent blocking system transported moist air from the North Atlantic towards the Alps (Yessimbet et al., 2022).
4.4 Challenges
Atmospheric blocking affects the occurrence and persistence of both very dry conditions and extreme precipitation across Europe. However, compared to the link between blocking and temperature extremes, the links between blocking and hydrometeorological extremes are more complex and modulated by local factors such as orography or proximity to moisture sources. Whether such local feedback substantially limit medium-range to sub-seasonal predictability of the blocking-related hydrometeorological extremes is an open question. Also a more systematic analysis of the link between blocking and flash floods in summer including the role of soil moisture would be important from a sub-seasonal predictability point of view.
5.1 Wind extremes and storm surges
Additional to its relevance for precipitation and temperature extremes (see Sects. 3 and 4), the presence of a blocking system can also be instrumental for the occurrence of wind extremes. Following Pfahl (2014), this influence can be distinguished in two main variants. First, as discussed in Sect. 3 the presence of a blocking system leads to the deflection of cyclones to the regions surrounding the system, resulting both in areas with high-wind extremes and calms (low-wind extremes). For example, given a high-latitude blocking system over the North Atlantic–European region, the jet stream and the storm track is shifted southward, corresponding to a negative phase of the NAO (Wanner et al., 2001). A good example of such a large-scale atmospheric setup is the winter 2009–2010, with a very persistent blocking system near Greenland which extended to Scandinavia around 60∘ N (Santos et al., 2013). This led to enhanced cyclone activity extending from the North Atlantic into the Mediterranean area and strong mean wind conditions around 45∘ N, while low cyclone numbers and reduced mean winds were found near Greenland and Iceland (Santos et al., 2013). In the winter 2011–2012, the opposite situation occurred: a very persistent blocking system was located over western Europe, around 50∘ N, 0∘ E (Santos et al., 2013). This was associated with a northward shift of the jet stream and a very positive NAO phase. In this case, cyclone numbers and mean wind speeds were very low around western and central Europe, while the number of cyclones and wind speed was enhanced near Greenland (Santos et al., 2013).
Second, blocking (or more precisely the associated surface high-pressure system) can be determinant to establish – together with a low-pressure anomaly – a very strong near-surface pressure gradient. A strong pressure gradient implies in turn strong geostrophic near-surface winds. A good example of such a situation is storm Kyrill in January 2007 (see Table 3), where the presence of an intense blocking system over southern Europe contributed to an unprecedented high-pressure gradient over the German Bight and Baltic Sea before the storm Kyrill crossed the area (Fink et al., 2009). With the passage of the cyclone over the area, the pressure gradients increased even further, leading to widespread strong winds and wind gusts over western and central Europe and significant impacts (Fink et al., 2009). Based on the ERA-Interim data set and thus a large number of events, Pfahl (2014) confirmed the above insights and provided evidence that both cyclones and blocking are often present during the occurrence of wind extremes in western and central Europe. While cyclones are generally located north/northeast of the affected location, blocking is typically found south or southwest of the location and thus opposite to the location of the cyclones. This setup is more consistent for wind extremes over central Europe and less consistent for southern Europe. In summary, Pfahl (2014) provides clear evidence for the importance of surface anticyclones and blocking for the occurrence of wind extremes.
Specifically for extreme wind situations affecting the Iberia Peninsula, Karremann et al. (2016) have defined a “hybrid type” atmospheric setup (Fig. 5), which is characterized by a strong pressure gradient over Iberia due to the juxtaposition of low- and high-pressure centers in the area. Such large-scale situations are often associated with an intensified jet stream, sometimes sustained by wave breaking on both flanks (e.g., Pinto et al., 2014; Messori and Caballero, 2015). The hybrid type corresponds to about 30 % of the cases for wind extremes affecting Iberia. The later study of Hénin et al. (2020) further documented the importance of the hybrid type cases for the occurrence of wind extremes in the region, though the relative number of extreme wind events associated with this large-scale setup is lower (about 15 %). Differences between both studies are primarily attributed to the different reanalysis data sets and periods analyzed.
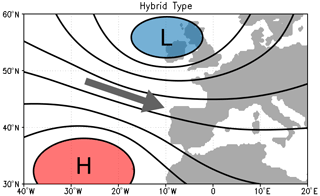
Figure 5Schematic illustration of the hybrid type pattern with a low-pressure system over the British Isles and a high-pressure system over the southern North Atlantic (inspired by Hénin et al., 2020).
Both persistent strong and persistent low-wind-speed conditions can have important impacts, for example, related to the energy production. Weber et al. (2019) analyzed the synoptic conditions leading to persistent (high or low) wind power production in the German Bight. Prolonged calms are primarily associated with nearby atmospheric blocking events, which can have a lifetime of up to several weeks. Still, this is not the case for all low-wind situations, and some variability in the weather patterns is identified. The predominant characteristic is that the longest cases are associated with very weak pressure gradients over the area and thus calms with low wind speeds and typically high persistence (Weber et al., 2019). This is in line with Grams et al. (2017), who analyzed the relationship between weather regimes and wind energy production in Europe. Regarding persistent strong-wind situations, they are typically associated with westerly flow and strong meridional pressure gradients. A new insight in this study is that high-wind periods may be distinctly longer than low-wind periods. This may be unexpected, as surface cyclones are typically more transient than high-pressure centers. However, and for at least one of the analyzed longer lasting periods, the temporal clustering of cyclones (Mailier et al., 2006; Pinto et al., 2014) has apparently played a crucial role in maintaining the strong-wind conditions for the extended period.
Coastal storm surges are typically associated with the passage of a cyclone (or cyclones) near coastal areas, which push the surface water masses towards the coast through wind stress (e.g., Dangendorf et al., 2016). In synoptic terms, these situations are often characterized by the presence of a long-lasting large-scale pressure gradient, which is then strengthened by the passing storm itself, thus leading to sustained winds and, in combination with the tides, to a high water levels in specific coastal areas. Analogously to the examples given above, a closer look reveals a large-scale setup with the juxtaposition of a high- and a low-pressure center, with the former often being associated with blocking. A notable example is the storm surge in the German Bight in December 2013 which was associated with the passage of storm Xaver (see Table 3) (Dangendorf et al., 2016). While Xaver passed over southern Scandinavia in an east-southeast path, the presence of a strong anticyclone over southwestern Europe created an impressive and long-enduring pressure gradient over the German Bight (Dangendorf et al., 2016; Staneva et al., 2016). This led to sustained north-northwesterly winds, resulting in the extraordinary storm surge characterized by record breaking sea levels in several coastal stretches in Lower Saxony (Jensen et al., 2015; Dangendorf et al., 2016). The presence (or not) of a long-lasting pressure gradient is crucial given that it is the combination/interaction of the surge and the tidal components (Horsburgh and Wilson, 2007) that leads to the actual coastal high waters – the longer the sustained large-scale winds (and thus the surge), the more probable high coastal water levels may be. Janjic et al. (2018) analyzed a number of storm surges affecting Ireland in the unprecedented storm winter of 2013–2014 (Matthews et al., 2014). Several of the analyzed cases (including the above-described storm Xaver; see Table 3) point to synoptic situations with a juxtaposition of a passing low-pressure center close to Scotland and the presence of a blocking system or an anticyclonic ridge to the south, thus inducting a strong pressure gradient. Focusing on the last 100 years, Haigh et al. (2016) investigated storm surges across the UK coasts. They stress the role of temporal cyclone clustering (Mailier et al., 2006; Pinto et al., 2014) for the longer surge events. Given the typical dynamical setup associated with cyclone clustering, it is assumed that high-pressure systems associated with long-lasting blocking systems at lower latitudes have contributed to the strong impacts in these situations.
5.2 Compound events
Compound events are defined as a “combination of multiple drivers and/or hazards that contribute to societal or environmental risk” (Zscheischler et al., 2018), according to the IPCC SREX (Leonard et al., 2014) and within the IPCC risk framework. In Zscheischler et al. (2020), a typology of compound events was proposed and analytical and modeling approaches were suggested to aid in the investigation of compound events. The four classes defined in Zscheischler et al. (2020) are
-
preconditioned, where a weather-driven or climate-driven precondition aggravates the impacts of a hazard;
-
multivariate, where multiple drivers and/or hazards lead to an impact;
-
temporally compounding, where a succession of hazards leads to an impact;
-
and spatially compounding, where hazards in multiple connected locations cause an aggregated impact.
Preconditioned compound events can also be connected to blocking activity. The example mentioned in Sect. 4 – a rain-on-snow event in October 2011 in northern Switzerland – was associated with strong northerly flow underneath a trough and the subsequent arrival of an atmospheric river, which resulted in substantial flooding in the region. A blocking pattern over the Atlantic was responsible for both the amplification of the downstream trough and the transport of high amounts of moisture over the Atlantic (Piaget et al., 2015). Indeed, most of the case studies which have been presented so far fall within the definition of compound events, being good examples of multivariate events (see Fig. 2). For instance, concurrent droughts and heat waves can occur on different timescales. On shorter timescales, compound hot and dry conditions are attributable to blocking (Röthlisberger and Martius, 2019) and to soil moisture–atmosphere interactions as mentioned in the different case studies (Fischer et al., 2007; Miralles et al., 2014). On longer timescales, compound dry winters/springs and hot summers seem to be occurring more often especially in the Mediterranean region, due to land–atmosphere interactions (Schumacher et al., 2019). Concurrent droughts and heat waves can cause additional and amplified impacts (e.g., wildfires, crop losses, natural vegetation death, losses in power plant operation, reduction of fisheries) (Zscheischler et al., 2020). Another important class of compound events that can be related to blocking patterns is temporally clustering events. In Sect. 5.1, a case of temporal cyclone clustering (Mailier et al., 2006; Pinto et al., 2014) that had impact on longer storm surges or even extreme precipitation is mentioned (Priestley et al., 2017). In these case studies, it is assumed that long-lasting blocking systems at lower latitudes have contributed to the strong impacts (see Sect. 5.1).
5.3 Challenges
In addition to temperature and hydrological extremes, wind extremes can also be influenced by blocking. This influence is primarily caused by changes in the horizontal pressure gradients and/or by a shift in the cyclone tracks. The same blocking system can lead to windless conditions in one place and stormy conditions in another. Moreover, blocking can also be responsible for the occurrence of so-called compound events. When multiple extremes are involved, the dynamical interactions within the flow environment of the blocking system become even more complex. This complexity poses a challenge to numerical weather prediction. Our understanding of the complex relationships between blocking, wind extremes and/or compound events would greatly benefit from targeted research activities.
The prediction of blocking is still a challenging task due to the complex character of these systems. In particular, this complexity is related to the interactions with other flow features (e.g., transient eddies) on different spatial and temporal scales. Nevertheless, blocking occurrence can also be a source of predictability in medium-range and subseasonal forecasts (e.g., Vitart et al., 2014; Quandt et al., 2017), which may benefit the predictions of related surface impacts. However, as shown in the previous sections (particularly the case studies), surface impacts of blocking systems can be highly variable, raising the question if there is an increased predictability for surface extremes caused by the occurrence of blocking compared to non-blocking situations.
Predictability of heat waves related to blocking on daily to seasonal timescales may, in principle, arise from the dynamics and persistence of the blocking as well as from soil–moisture feedbacks. Blocking formation and maintenance are mainly driven by atmospheric processes (see Sect. 2) with characteristic timescales and thus potential predictability of several days to 1–2 weeks but can also be influenced by boundary conditions such as sea surface temperatures and teleconnections offering potential predictability on longer timescales (Trenberth and Fasullo, 2012; O'Reilly et al., 2016; Ferranti et al., 2018). The predictability of blocking is generally higher in its maintenance phase compared to blocking onset and decay (Tibaldi and Molteni, 1990; Reynolds et al., 2019). Soil moisture conditions are sensitive to precipitation accumulation over the preceding months and may thus yield predictability on seasonal and even multi-year timescales (Quesada et al., 2012; Breil et al., 2019). Altogether, there are indications that these processes related to blocking persistence and soil moisture give rise to a higher subseasonal predictability of heat extremes compared to average summers (Wulff and Domeisen, 2019). The linkage to blocking also provides opportunities for skillful statistical forecasts of heat waves (Chattopadhyay et al., 2020). Detailed studies of the predictability of the Russian heat wave in 2010 (Matsueda, 2011; Quandt et al., 2017, 2019) showed that the predictability of the blocking systems was generally high, in particular with respect to their onset and maintenance. Lower predictability was associated with the decay of the main blocking system and some details of the blocking characteristics (Quandt et al., 2017). These predictability differences were linked to upstream Rossby wave dynamics and moisture transport (Quandt et al., 2019).
Cold spells have a significant predictability within a 2-week lead time. However, a strong decrease during the first week of forecasts is noted, together with a generally reduced predictability during their onset and end phases (Lavaysse et al., 2019). Ferranti et al. (2018) investigated the impact of large-scale flow patterns and their transitions on the predictability of cold conditions over Europe. In this study, they introduce a novel framework dealing with the transition of (non-)blocking and NAO (±) situations (NAO–blocking phase space). They applied their method to reanalysis data and could show that NAO+ favors transitions into blocking, while blocking itself favors transitions into NAO−. They further investigated extended-range multi-model ensemble forecasts and found that the predictability of severe cold events depends on the type of transitions. For example, the extended-range ECMWF ensemble shows increased predictability of cold spells associated with the development of Greenland blocking. The forecast variability in the late winter cold spell in March 2018 (see Sect. 3) was investigated by Kautz et al. (2020). The analysis of ECMWF sub-seasonal ensemble forecasts could show that the occurrence of a Scandinavian blocking anticyclone at the end of February as well as the regime shift to a strong NAO− phase influenced the predictability of the cold spell. Ensemble members which showed the NAO− pattern also captured the cold spell. On the other hand, members which additionally captured the precursor blocking over Scandinavia featured a better representation of the southeastern extension of the cold spell. Besides studies which investigated the relation between cold spell and blocking predictability in operational models, there are also efforts to produce skillful seasonal predictions of winter blocking and temperature extremes with the help of statistical models. For example Miller and Wang (2019) developed a multiple linear regression model using predictors based on sea surface temperature, 70 hPa geopotential height and sea ice concentration which skillfully predicts the blocking frequency over Eurasia. In addition, they developed similar models also addressing the relationship between blocking and surface temperature extremes. With these models, cold anomalies over Eurasia and Greenland would be skillfully predicted.
While there are a few studies which connect the predictability of temperature extremes on the medium range and the sub-seasonal timescale with the occurrence of blocking, there are hardly any comparable studies which focus specifically on the predictability of wind or rain extremes in connection with blocking. An exception is studies that relate the predictability of wind extremes to the NAO (which is strongly connected to blocking; see Sect. 3). Scaife et al. (2014) have shown that winter wind predictions over Europe benefit form the influences of the NAO, meaning that strong-wind events are poorly predicted in regions where the NAO influence is weak. However, there is a lack of studies that examine the relationship between the predictability of low- and high-wind events and blocking directly. This could be a perspective for future research, especially as skillful predictions of wind extremes are highly relevant for the renewable energy sector (e.g., Beerli et al., 2017). At the eastern flank of the blocking system, which was associated with the Russian heat wave in 2010, heavy rain events led to flooding in Pakistan (e.g., Lau and Kim, 2012). While the impact of the blocking on the predictability of the heat wave was investigated (Matsueda, 2011; Quandt et al., 2017), it is less clear how blocking has influenced the prediction of the extreme rain in Pakistan. There are only studies which deal with the predictability of the Pakistan floods without any linkage to blocking (Webster et al., 2011). Another example is the 2013 flooding event in Europe. Ionita et al. (2015) investigated its predictability by using a multiple linear regression model. They could show that an accurate prediction of the June 2013 Elbe river extreme discharge was possible by considering the amount of precipitation in May and June as well as May soil moisture and sea level pressure. They did not use blocking as a predictor for their model. However, they also discussed the synoptic flow pattern and emphasized that the persistent blocking system which evolved in mid-May over Scandinavia could have been already considered an indicator for a potential flood.
The occurrence and persistence of blocking are underestimated in climate models (see Sect. 2) (e.g., Davini and D'Andrea, 2016). A recent study shows that seasonal forecasts are suitable for analyzing the blocking bias in numerical models, which can help to improve climate models (Davini et al., 2021). The underestimation of frequency varies with the region of occurrence and the season (Davini and D'Andrea, 2016). For example, the underestimation of Atlantic/European blocking is lower in summer than in winter (Woollings et al., 2018), which is relevant for the prediction of surface extremes. Studies show that despite the bias, the link between extreme temperatures and blocking can be captured in climate models (Schaller et al., 2018). This is particularly important for heat waves in summer. Nevertheless, as for predictions in the medium and (sub-)seasonal range, the predictability of blocking in climate models cannot be transferred one to one to the predictability of surface extremes. In the next section, we discuss the relation between blocking and weather extremes in a warming climate.
The relevance of extreme weather events for society may increase in the coming years, as regional changes in the magnitude and frequency of these events are expected due to global warming (Mitchell et al., 2006; Rahmstorf and Coumou, 2011; Coumou and Rahmstorf, 2012; Mitchell et al., 2016). Changes in the characteristics of several of these extreme events have already been detected in observations over the last decades (Folland et al., 2002; Sparks and Menzel, 2002; Rahmstorf et al., 2012) and are evident in climate projections for the near and distant future (Kjellström et al., 2011; Branković et al., 2012). As blocking systems can trigger weather extremes, it is also of interest how blocking characteristics may change in the future and how these changes can in turn influence the occurrence and characteristics of surface extreme weather events.
Future projections show generally a decrease in blocking frequency over the mid-latitudes, but there are hints at an increase in blocking duration (Sillmann and Croci-Maspoli, 2009; Davini and D'Andrea, 2020). However, changes in blocking occurrence cannot be generalized for the entire Northern Hemisphere, as there are strong regional differences. For example, the frequency and duration of Ural blocking is projected to increase under future climate conditions (Dunn-Sigouin and Son, 2013; Luo et al., 2018; Davini and D'Andrea, 2020). In contrast, the frequency of blocking systems over the Euro-Atlantic sector shows a significant decrease in climate simulations in future decades, independent of the considered blocking duration (Matsueda et al., 2009; Masato et al., 2013b). This decrease is focused on the western flank of these blocking systems, whereas an increase is predicted on their eastern flank, indicating an overall shift of blocking activity towards Eurasia (in the same location where the 2010 Russian heat wave blocking was observed) (Matsueda et al., 2009; Masato et al., 2013b; Davini and D'Andrea, 2020). A shift in location is expected not only zonally but also meridionally: a poleward shift of blocking activity in summer indicates that there will be more high-latitude blocking during this season but less in the mid-latitudes (Masato et al., 2013b; Matsueda and Endo, 2017). Moreover, the size of blocking systems over the Northern Hemisphere is projected to increase with climate change (Nabizadeh et al., 2019), but no noticeable hemispheric changes are expected in blocking duration (Dunn-Sigouin and Son, 2013). The expected changes in blocking depend on several factors, including stratospheric variability, changes in the mid-latitude jet stream with respect to intensity as well as location, and near-surface Arctic warming (Francis and Vavrus, 2015; Kennedy et al., 2016).
The expected future changes in blocking might also influence characteristics of future European heat waves. Climate models project a general increase in extreme summer temperatures and heat wave intensity (Meehl and Tebaldi, 2004; Fischer and Schär, 2010; Perkins et al., 2012) that is mainly related to the thermodynamic effects of climate warming. Under future climate conditions, blocking is projected to remain the most relevant circulation feature initiating European heat waves (Brunner et al., 2018; Schaller et al., 2018). Despite some indications of a weakening of the midlatitude circulation in summer in recent decades (Coumou et al., 2015; Horton et al., 2015), studies on future changes in the properties of weather systems associated with heat waves (such as blocking persistence) come to diverging conclusions (Plavcova and Kysely, 2013; Brunner et al., 2018; Mann et al., 2018; Schaller et al., 2018; Huguenin et al., 2020; Jézéquel et al., 2020). Whether future changes in blocking dynamics might lead to changes in heat waves beyond their thermodynamic intensification thus remains an important question for future research. Given that the new CMIP6 generation of complex earth system models has a higher resolution and an improved representation of physical processes, also regarding small-scale processes and extreme events (Chen et al., 2022), advances in this area can be expected in the next few years.
The relation between blocking and low-temperature extremes will remain relevant in the future. Under future climate conditions, the presence of long-lasting blocking systems over the North Atlantic increases the probability of low surface temperatures over the European continent in winter (Sillmann et al., 2011). Climate projections show that western European cold spells will become comparatively warmer and may partly remain above the freezing point under future climate conditions (de Vries et al., 2012). These changes in cold spell characteristics can be partly associated with changes in blocking, whereas changes in other large-scale patterns (zonal temperature gradient and strength of the westerlies) have an additional contribution (Screen, 2014). For example, thermal advection leading to cold extremes in winter could weaken as a result of the weakened mean temperature gradients (Kennedy et al., 2016).
Regarding precipitation, negative anomalies (associated with dry conditions) around the British Isles as well as positive anomalies (associated with wet conditions) along the southeastern coast of Greenland and over parts of the North Atlantic are related to European blocking (Sillmann and Croci-Maspoli, 2009). Climate projections show that these patterns will increase in a warming climate. The increase in positive precipitation anomalies over the North Atlantic is related to the passage of cyclones at the southern flank of the blocking systems.
The changes in blocking features (such as frequency) can affect the occurrence of extreme surface weather. However, since the occurrence of such extreme weather events is also influenced by other factors (e.g., thermodynamical factors), it is not possible to transfer the changes in blocking one to one (Woollings et al., 2018; Nabizadeh et al., 2021).
In the previous sections we have provided an overview on the relationship between the occurrence of blocking and different types of extreme events. This said, it is important to note that not every blocking system leads to the occurrence of an extreme weather event and that extreme weather events can also be favored by other large-scale flow patterns (such as an intense zonal flow) (e.g., Trigo et al., 2004; Priestley et al., 2017) and strongly influenced by local effects (such as orography) (e.g., Hofherr and Kunz, 2010). In particular, the persistence of blocking alone is not necessarily a meaningful indication that an extreme event will actually occur. The spatial component also plays a decisive role; i.e., the actual location of the blocking system is crucial for the formation and type of extreme event. In this review, we have shown that blocking systems are capable of triggering a variety of extreme events (e.g., heat waves or flooding), sometimes even directly following each other at the same location or at the same time (known under the term compound events). Based on the above, several research gaps have been identified, for which research perspectives are given below.
The dynamic relationships between extreme weather events and blocking are generally not fully understood. This is also due to the fact that the dynamics of blocking systems are complex and cover a wide range of spatial scales and timescales. Among other reasons, this is due to the partly nonlinear interactions with the large-scale flow as well as with other weather systems such as transient eddies (e.g., Shutts, 1983). In addition to the interactions within the troposphere, there are feedback mechanisms with the land masses and the oceans as well as coupling processes with the stratosphere (e.g., Woollings et al., 2010). Especially the latter are not yet completely understood.
The results on (statistically significant) correlations between two phenomena depend on the choice of detection methods, which is also true for blocking and weather extremes. Of the approximately 290 studies referenced in our article, 45 % deal directly with weather extremes associated with blocking, and there are twice as many studies of temperature anomalies than of hydrological events. Of the studies that address weather extremes associated with blocking, 42 % do not use a blocking index. Instead, most of rely on synoptic descriptions or refer to other studies that have identified the responsible system as blocking – 41 % of these are case studies. Of the studies that discuss weather extremes associated with blocking, 58 % use a blocking index – only 27 % of these present case studies, which is probably related to the fact that a detection method is mandatory for analysis of long data sets and to derive statistical correlations. In the studies reported here, different blocking indices (see Sect. 2) were used for both hydrological and temperature extreme events, which shows that the relations between blocking and these types of extremes can usually be observed independently of the choice of blocking index. Nevertheless, there is a dependence on the choice of index, as described in Sect. 3 for heat wave and as investigated in Chan et al. (2019). The location of the blocking system also depends on the type of detection method (e.g., anomaly vs. reversal of gradient), which is particularly relevant for the phasing between blocking and weather extremes (Doblas-Reyes et al., 2002). Therefore, an investigation of the extent to which the choice of blocking index plays a role in the analysis of different types of extremes is an interesting question for further research.
Through field campaigns, data on blocking systems and/or on associated extremes can typically be collected only over a small area but at a high temporal and spatial resolution (e.g., 2016 NAWDEX block case study – Maddison et al., 2019; Steinfeld et al., 2020; or the 2003 UK TORCH campaign – Vieno et al., 2010). Long-term measurements, both in situ and from remote sensing systems, also provide important data when outliers (e.g., temperature records) are captured (Brunner et al., 2017). The analysis of such data can help to improve our understanding, for example, as input for further simulation studies. With respect to coupling processes between the troposphere and stratosphere, which can influence blocking and thus also extreme weather events, there is a lack of such long-term measurements. The potential of satellite data for blocking analysis has been shown in Brunner et al. (2016) and Brunner and Steiner (2017). They show for high-impact blocking events (e.g., the blocking system associated with the 2010 Russian heat wave) that GPS radio occultation observations can be used to capture the vertical structure of blocking systems.
A better understanding of the dynamics through more and better observations would also have a positive effect on the capturing of blocking systems and their interactions in numerical models. Currently, the representation of blocking in numerical weather and climate models has several weaknesses regarding its frequency, intensity and persistence. If we look explicitly at the life cycle of blocking systems, the onset and the decay phases are the periods that pose the primary challenge for numerical models (e.g., Frederiksen et al., 2004). If the blocking system is part of the initial conditions in a weather forecast, this can have a positive effect on the predictability of surface conditions (also on sub-seasonal timescales) due to the potential persistence of the blocking system. However, because blocking decay is difficult to predict, the duration of blocking systems is also often incorrectly predicted. It is precisely the poor predictability of the decay of the blocking system that inevitably transfers to or at least influences the predictability of the decay of an extreme weather event (e.g., Quandt et al., 2017). Nevertheless, recent studies have used the relations between blocking and temperature extremes and provided evidence of improved predictability for the surface conditions (Ferranti et al., 2018). However, there are hardly any comparable studies which focus specifically on the predictability of wind extremes or heavy precipitation in connection with blocking. Further studies are thus needed to quantify the potential of these relations for weather forecasting, decadal predictions and climate projections.
When considering the relationships between blocking systems and extreme events, we are pursuing a moving target as both decadal variability and long-term global climate change modulate the occurrence of both blocking and extreme weather events. We arguably have a very limited sample of observed extreme events relevant to today's climate. The consideration of larger samples of data (Maher et al., 2019; Ehmele et al., 2020) is thus of key importance to provide robust estimates of these relationships in a changing climate. Whether future changes in blocking dynamics might lead to changes in heat waves beyond their thermodynamic intensification thus remains an important question for future research. In this line of research, attribution studies can provide another important piece of the puzzle (Stott et al., 2016; Swain et al., 2020; van Garderen et al., 2021). Therefore, further studies are needed to understand and estimate the relation between blocking and weather extremes under climate change.
Further studies on blocking and their influence on weather extremes are thus needed to understand the underlying physical mechanisms. Both observational data sets, which provide an important data basis, and new modeling strategies can provide a possible perspective to improve this understanding as well as the predictability and risk assessment of extreme weather events. Potential changes in weather extremes due to global climate warming also increase the need for better forecasting and risk assessment. For planned observational campaigns as well as long-term observations, feedback and coupling processes should be covered in particular. The same applies to model experiments, for example realized in seamless modeling approaches. The framework presented in this review article could also serve as a reference to align future studies in this complex topic, thus enabling a stronger inter-study comparability.
In the study, we used two ERA5 data sets (Hersbach et al., 2020): (1) ERA5 monthly averaged data on single levels from 1979 to present (Hersbach et al., 2019a, https://doi.org/10.24381/cds.f17050d7) and (2) ERA5 monthly averaged data on pressure levels from 1979 to present (Hersbach et al., 2019b, https://doi.org/10.24381/cds.6860a573).
The original idea for the paper was developed by LAK and JGP. The concept was developed together with all co-authors. LAK wrote the introduction (Sect. 1); the parts addressing cold spells (Sect. 3); and the sections about predictability, climate change and research perspectives (Sects. 6–8). TW wrote the background section (Sect. 2). SP wrote the passages on heat waves (Sect. 3) and contributed to Sects. 6 and 7. In Sect. 4, OM wrote the parts on heavy precipitation, flood events and extreme snowfall, while AMR and PMS wrote the parts addressing droughts. In Sect. 5, JGP wrote the parts on wind extremes and AMR on compound events. The figures were prepared by LAK, with the idea for Fig. 2 coming from OM. All authors contributed with discussions and revisions.
At least one of the (co-)authors is a member of the editorial board of Weather and Climate Dynamics. The peer-review process was guided by an independent editor, and the authors also have no other competing interests to declare.
Publisher's note: Copernicus Publications remains neutral with regard to jurisdictional claims in published maps and institutional affiliations.
We thank the ECMWF for access to the ERA5 reanalyses. Pedro M. Sousa would like to thank project HOLMODRIVE (PTDC/CTA-GEO/29029/2017), funded through FCT (Fundação para a Ciência e a Tecnologia, Portugal). Alexandre M. Ramos acknowledges the support of the Fundação para a Ciência e a Tecnologia of Portugal (FCT) through the project WEx-Atlantic (PTDC/CTAMET/29233/2017) and Scientific Employment Stimulus 2017 (CEECIND/00027/2017). Olivia Martius acknowledges support from the Swiss National Science Foundation grant no. 178751. Joaquim G. Pinto thanks the AXA Research Fund for support. Lisa-Ann Kautz and Joaquim G. Pinto thank the Transregional Collaborative Research Center SFB/TRR 165 “Waves to Weather” funded by the German Research Foundation (DFG) for support. Lisa-Ann Kautz and Joaquim G. Pinto thank Susanna Mohr and Christian Grams (both KIT) for helpful discussions.
This research has been supported by FCT (Fundação para a Ciência e a Tecnologia, Portugal) (HOLMODRIVE, grant no. PTDC/CTA-GEO/29029/2017; WEx-Atlantic, grant no. PTDC/CTAMET/29233/2017; Scientific Employment Stimulus 2017, grant no. CEECIND/00027/2017), the Swiss National Science Foundation (grant no. 178751), the Deutsche Forschungsgemeinschaft (grant no. SFB/TRR 165, Transregional Collaborative Research Center “Waves to Weather”) and the AXA Research Fund (AXA Chair “Regional Climate and Weather Hazards”).
This paper was edited by Pedram Hassanzadeh and reviewed by Noboru Nakamura and two anonymous referees.
Alfieri, L., Dottori, F., Betts, R., Salamon, P., and Feyen, L.: Multi-model projections of river flood risk in Europe under global warming, Climate, 6, 6, https://doi.org/10.3390/cli6010006, 2018. a
Altenhoff, A. M., Martius, O., Croci-Maspoli, M., Schwierz, C., and Davies, H. C.: Linkage of atmospheric blocks and synoptic-scale Rossby waves: a climatological analysis, Tellus A, 60, 1053–1063, 2008. a
Anagnostopoulou, C., Tolika, K., Lazoglou, G., and Maheras, P.: The exceptionally cold January of 2017 over the Balkan Peninsula: A climatological and synoptic analysis, Atmosphere, 8, 252, https://doi.org/10.3390/atmos8120252, 2017. a, b, c, d
Aon: Weather, Climate and Catastrophe Insight – 2017 Annual Report, http://thoughtleadership.aon.com/Documents/20180124-ab-if-annual-report-weather-climate-2017.pdf (last access: 21 November 2021), 2018. a
Athanasiadis, P. J., Bellucci, A., Hermanson, L., Scaife, A. A., MacLachlan, C., Arribas, A., Materia, S., Borrelli, A., and Gualdi, S.: The representation of atmospheric blocking and the associated low-frequency variability in two seasonal prediction systems, J. Climate, 27, 9082–9100, https://doi.org/10.1175/JCLI-D-14-00291.1, 2014. a
Athanasiadis, P. J., Yeager, S., Kwon, Y.-O., Bellucci, A., Smith, D. W., and Tibaldi, S.: Decadal predictability of North Atlantic blocking and the NAO, npj Climate and Atmospheric Science, 3, 1–10, https://doi.org/10.1038/s41612-020-0120-6, 2020. a
Athanasopoulou, E., Rieger, D., Walter, C., Vogel, H., Karali, A., Hatzaki, M., Gerasopoulos, E., Vogel, B., Giannakopoulos, C., Gratsea, M., and Roussos, A.: Fire risk, atmospheric chemistry and radiative forcing assessment of wildfires in eastern Mediterranean, Atmos. Environ., 95, 113–125, https://doi.org/10.1016/j.atmosenv.2014.05.077, 2014. a
Austin, J.: The blocking of middle latitude westerly winds by planetary waves, Q. J. Roy. Meteor. Soc., 106, 327–350, https://doi.org/10.1002/qj.49710644807, 1980. a
Barnes, E. A., Slingo, J., and Woollings, T.: A methodology for the comparison of blocking climatologies across indices, models and climate scenarios, Clim. Dynam., 38, 2467–2481, https://doi.org/10.1007/s00382-011-1243-6, 2012. a
Barriopedro, D., García-Herrera, R., Lupo, A. R., and Hernández, E.: A climatology of Northern Hemisphere blocking, J. Climate, 19, 1042–1063, https://doi.org/10.1175/JCLI3678.1, 2006. a, b, c
Barriopedro, D., García-Herrera, R., and Trigo, R. M.: Application of blocking diagnosis methods to general circulation models. Part I: A novel detection scheme, Clim. Dynam., 35, 1373–1391, https://doi.org/10.1007/s00382-010-0767-5, 2010. a
Barriopedro, D., Fischer, E. M., Luterbacher, J., Trigo, R. M., and García-Herrera, R.: The Hot Summer of 2010: Redrawing the Temperature Record Map of Europe, Science, 332, 220–224, https://doi.org/10.1126/science.1201224, 2011. a, b, c, d, e
Bastos, A., Ciais, P., Friedlingstein, P., Sitch, S., Pongratz, J., Fan, L., Wigneron, J. P., Weber, U., Reichstein, M., Fu, Z., Anthoni, P., Arneth, A., Haverd, V., Jain, A. K., Joetzjer, E., Knauer, J., Lienert, S., Loughran, T., McGuire, P. C., Tian, H., Viovy, N., and Zaehle, S.: Direct and seasonal legacy effects of the 2018 heat wave and drought on European ecosystem productivity, Sci. Adv., 6, eaba2724, https://doi.org/10.1126/sciadv.aba2724, 2020. a
Beerli, R., Wernli, H., and Grams, C. M.: Does the lower stratosphere provide predictability for month-ahead wind electricity generation in Europe?, Q. J. Roy. Meteor. Soc., 143, 3025–3036, https://doi.org/10.1002/qj.3158, 2017. a
Bengtsson, L.: Numerical prediction of atmospheric blocking – A case study, Tellus, 33, 19–42, https://doi.org/10.3402/tellusa.v33i1.10692, 1981. a
Beniston, M. and Diaz, H. F.: The 2003 heat wave as an example of summers in a greenhouse climate? Observations and climate model simulations for Basel, Switzerland, Global Planet. Change, 44, 73–81, https://doi.org/10.1016/j.gloplacha.2004.06.006, 2004. a, b
Berckmans, J., Woollings, T., Demory, M.-E., Vidale, P.-L., and Roberts, M.: Atmospheric blocking in a high resolution climate model: influences of mean state, orography and eddy forcing, Atmos. Sci. Lett., 14, 34–40, https://doi.org/10.1002/asl2.412, 2013. a, b
Berggren, R., Bolin, B., and Rossby, C.-G.: An aerological study of zonal motion, its perturbations and break-down, Tellus, 1, 14–37, https://doi.org/10.1111/j.2153-3490.1949.tb01257.x, 1949. a, b, c
Bieli, M., Pfahl, S., and Wernli, H.: A Lagrangian investigation of hot and cold temperature extremes in Europe, Q. J. Roy. Meteor. Soc., 141, 98–108, https://doi.org/10.1002/qj.2339, 2015. a, b, c, d
Black, E., Blackburn, M., Harrison, G., Hoskins, B., and Methven, J.: Factors contributing to the summer 2003 European heatwave, Weather, 59, 217–223, https://doi.org/10.1256/wea.74.04, 2004. a, b, c, d
Blöschl, G., Nester, T., Komma, J., Parajka, J., and Perdigão, R. A. P.: The June 2013 flood in the Upper Danube Basin, and comparisons with the 2002, 1954 and 1899 floods, Hydrol. Earth Syst. Sci., 17, 5197–5212, https://doi.org/10.5194/hess-17-5197-2013, 2013. a, b, c, d
Booth, J. F., Dunn-Sigouin, E., and Pfahl, S.: The Relationship Between Extratropical Cyclone Steering and Blocking Along the North American East Coast, Geophys. Res. Lett., 44, 11976–11984, https://doi.org/10.1002/2017GL075941, 2017. a
Branković, Č., Patarčić, M., Güttler, I., and Srnec, L.: Near-future climate change over Europe with focus on Croatia in an ensemble of regional climate model simulations, Clim. Res., 52, 227–251, https://doi.org/10.3354/cr01058, 2012. a
Breil, M., Laube, N., Pinto, J. G., and Schädler, G.: The impact of soil initialization on regional decadal climate predictions in Europe, Clim. Res., 77, 139–154, https://doi.org/10.3354/cr01548, 2019. a
Brunner, L. and Steiner, A. K.: A global perspective on atmospheric blocking using GPS radio occultation – one decade of observations, Atmos. Meas. Tech., 10, 4727–4745, https://doi.org/10.5194/amt-10-4727-2017, 2017. a
Brunner, L., Steiner, A. K., Scherllin-Pirscher, B., and Jury, M. W.: Exploring atmospheric blocking with GPS radio occultation observations, Atmos. Chem. Phys., 16, 4593–4604, https://doi.org/10.5194/acp-16-4593-2016, 2016. a
Brunner, L., Hegerl, G. C., and Steiner, A. K.: Connecting atmospheric blocking to European temperature extremes in spring, J. Climate, 30, 585–594, https://doi.org/10.1175/JCLI-D-16-0518.1, 2017. a
Brunner, L., Schaller, N., Anstey, J., Sillmann, J., and Steiner, A. K.: Dependence of Present and Future European Temperature Extremes on the Location of Atmospheric Blocking, Geophys. Res. Lett., 45, 6311–6320, https://doi.org/10.1029/2018GL077837, 2018. a, b, c, d, e
Buehler, T., Raible, C. C., and Stocker, T. F.: The relationship of winter season North Atlantic blocking frequencies to extreme cold or dry spells in the ERA-40, Tellus A, 63, 174–187, https://doi.org/10.1111/j.1600-0870.2010.00492.x, 2011. a, b, c
Cassou, C., Terray, L., and Phillips, A. S.: Tropical Atlantic Influence on European Heat Waves, J. Climate, 18, 2805–2811, https://doi.org/10.1175/JCLI3506.1, 2005. a, b
Cattiaux, J., Vautard, R., Cassou, C., Yiou, P., Masson-Delmotte, V., and Codron, F.: Winter 2010 in Europe: A cold extreme in a warming climate, Geophys. Res. Lett., 37, L20704, https://doi.org/10.1029/2010GL044613, 2010. a, b, c, d
Chan, P.-W., Hassanzadeh, P., and Kuang, Z.: Evaluating Indices of Blocking Anticyclones in Terms of Their Linear Relations With Surface Hot Extremes, Geophys. Res. Lett., 46, 4904–4912, https://doi.org/10.1029/2019GL083307, 2019. a, b, c
Charlton-Perez, A. J., Aldridge, R. W., Grams, C. M., and Lee, R.: Winter pressures on the UK health system dominated by the Greenland Blocking weather regime, Weather and Climate Extremes, 25, 100218, https://doi.org/10.1016/j.wace.2019.100218, 2019. a
Chattopadhyay, A., Nabizadeh, E., and Hassanzadeh, P.: Analog Forecasting of Extreme-Causing Weather Patterns Using Deep Learning, J. Adv. Model. Earth Syst., 12, e2019MS001958, https://doi.org/10.1029/2019MS001958, 2020. a
Chen, D., Rojas, M., Samset, B. H., Cobb, K., Diongue Niang, A., Edwards, P., Emori, S., Faria, S. H., Hawkins, E., Hope, P., Huybrechts, P., Meinshausen, M., Mustafa, S. K., Plattner, G.-K., and Tréguier, A.-M.: Framing, Context, and Methods, in: Climate Change 2021: The Physical Science Basis, Contribution of Working Group I to the Sixth Assessment Report of the Intergovernmental Panel on Climate Change, edited by: Masson-Delmotte, V., Zhai, P., Pirani, A., Connors, S. L., Péan, C., Berger, S., Caud, N., Chen, Y., Goldfarb, L., Gomis, M. I., Huang, M., Leitzell, K., Lonnoy, E., Matthews, J. B. R., Maycock, T. K., Waterfield, T., Yelekçi, O., Yu, R., and Zhou, B., Cambridge University Press, in press, 2022. a
Chen, G., Lu, J., Burrows, D. A., and Leung, L. R.: Local finite-amplitude wave activity as an objective diagnostic of midlatitude extreme weather, Geophys. Res. Lett., 42, 10–952, https://doi.org/10.1002/2015GL066959, 2015. a
Chen, K., Gawarkiewicz, G., Kwon, Y.-O., and Zhang, W. G.: The role of atmospheric forcing versus ocean advection during the extreme warming of the Northeast US continental shelf in 2012, J. Geophys. Res.-Oceans, 120, 4324–4339, https://doi.org/10.1002/2014JC010547, 2015. a
Cheung, H. N., Zhou, W., Shao, Y., Chen, W., Mok, H. Y., and Wu, M. C.: Observational climatology and characteristics of wintertime atmospheric blocking over Ural–Siberia, Clim. Dynam., 41, 63–79, https://doi.org/10.1007/s00382-012-1587-6, 2013. a
Colucci, S. J.: Explosive cyclogenesis and large-scale circulation changes: Implications for atmospheric blocking, J. Atmos. Sci., 42, 2701–2717, https://doi.org/10.1175/1520-0469(1985)042<2701:ECALSC>2.0.CO;2, 1985. a, b
Coumou, D. and Rahmstorf, S.: A decade of weather extremes, Nat. Clim. Change, 2, 491–496, https://doi.org/10.1038/nclimate1452, 2012. a
Coumou, D., Lehmann, J., and Beckmann, J.: The weakening summer circulation in the Northern Hemisphere mid-latitudes, Science, 348, 324–327, https://doi.org/10.1126/science.1261768, 2015. a
Croci-Maspoli, M. and Davies, H. C.: Key Dynamical Features of the 2005/06 European Winter, Mon. Weather Rev., 137, 664–678, https://doi.org/10.1175/2008MWR2533.1, 2009. a
Croci-Maspoli, M., Schwierz, C., and Davies, H. C.: Atmospheric blocking: Space-time links to the NAO and PNA, Clim. Dynam., 29, 713–725, 2007. a
Crum, F. X. and Stevens, D. F.: A case study of atmospheric blocking using isentropic analysis, Mon. Weather Rev., 116, 223–241, https://doi.org/10.1175/1520-0493(1988)116<0223:ACSOAB>2.0.CO;2, 1988. a, b
Dangendorf, S., Arns, A., Pinto, J. G., Ludwig, P., and Jensen, J.: The exceptional influence of storm “Xaver” on design water levels in the German Bight, Environ. Res. Lett., 11, 054001, https://doi.org/10.1088/1748-9326/11/5/054001, 2016. a, b, c, d, e
Davini, P. and D'Andrea, F.: Northern Hemisphere atmospheric blocking representation in global climate models: Twenty years of improvements?, J. Climate, 29, 8823–8840, https://doi.org/10.1175/JCLI-D-16-0242.1, 2016. a, b
Davini, P. and D'Andrea, F.: From CMIP-3 to CMIP-6: Northern Hemisphere atmospheric blocking simulation in present and future climate, J. Climate, 33, 10021–10038, https://doi.org/10.1175/JCLI-D-19-0862.1, 2020. a, b, c, d, e
Davini, P., Cagnazzo, C., Neale, R., and Tribbia, J.: Coupling between Greenland blocking and the North Atlantic Oscillation pattern, Geophys. Res. Lett., 39, L14701, https://doi.org/10.1029/2012GL052315, 2012. a
Davini, P., Weisheimer, A., Balmaseda, M., Johnson, S. J., Molteni, F., Roberts, C. D., Senan, R., and Stockdale, T. N.: The representation of winter Northern Hemisphere atmospheric blocking in ECMWF seasonal prediction systems, Q. J. Roy. Meteor. Soc., 147, 1344–1363, https://doi.org/10.1002/qj.3974, 2021. a
Davolio, S., Stocchi, P., Benetazzo, A., Bohm, E., Riminucci, F., Ravaioli, M., Li, X.-M., and Carniel, S.: Exceptional Bora outbreak in winter 2012: Validation and analysis of high-resolution atmospheric model simulations in the northern Adriatic area, Dynam. Atmos. Oceans, 71, 1–20, https://doi.org/10.1016/j.dynatmoce.2015.05.002, 2015. a
De Bono, A., Peduzzi, P., Kluser, S., and Giuliani, G.: Impacts of summer 2003 heat wave in Europe, United Nations Environment Programme, https://archive-ouverte.unige.ch/unige:32255 (last access: 19 November 2021), 2004. a
de'Donato, F. K., Leone, M., Noce, D., Davoli, M., and Michelozzi, P.: The impact of the February 2012 cold spell on health in Italy using surveillance data, PloS one, 8, e61720, https://doi.org/10.1371/journal.pone.0061720, 2013. a
Demirtaş, M.: The large-scale environment of the European 2012 high-impact cold wave: prolonged upstream and downstream atmospheric blocking, Weather, 72, 297–301, https://doi.org/10.1002/wea.3020, 2017. a, b, c, d, e, f
de Vries, H., Haarsma, R. J., and Hazeleger, W.: Western European cold spells in current and future climate, Geophys. Res. Lett., 39, L04706, https://doi.org/10.1029/2011GL050665, 2012. a
de Vries, H., van Westrhenen, R., and van Oldenborgh, G. J.: The February 2012 European cold spell that didn't bring the dutch another 11-city tour, in: Explaining Extreme Events of 2012 from a Climate Perspective, B. Am. Meteorol. Soc., 94, S26–S28, 2013. a, b
Doblas-Reyes, F. J., Casado, M., and Pastor, M.: Sensitivity of the Northern Hemisphere blocking frequency to the detection index, J. Geophys. Res.-Atmos., 107, ACL 6-1–ACL 6-22, https://doi.org/10.1029/2000JD000290, 2002. a
Donat, M. G., Pardowitz, T., Leckebusch, G. C., Ulbrich, U., and Burghoff, O.: High-resolution refinement of a storm loss model and estimation of return periods of loss-intensive storms over Germany, Nat. Hazards Earth Syst. Sci., 11, 2821–2833, https://doi.org/10.5194/nhess-11-2821-2011, 2011. a
Drouard, M. and Woollings, T.: Contrasting mechanisms of summer blocking over western Eurasia, Geophys. Res. Lett., 45, 12040–12048, https://doi.org/10.1029/2018GL079894, 2018. a, b, c
Drouard, M., Woollings, T., Sexton, D. M., and McSweeney, C. F.: Dynamical differences between short and long blocks in the Northern Hemisphere, J. Geophys. Res.-Atmos., 126, e2020JD034082, https://doi.org/10.1029/2020JD034082, 2021. a
Dunn-Sigouin, E. and Son, S.-W.: Northern Hemisphere blocking frequency and duration in the CMIP5 models, J. Geophys. Res.-Atmos., 118, 1179–1188, https://doi.org/10.1002/jgrd.50143, 2013. a, b
DWD: Cold spell in Europe and Asia in late winter 2011/2012, https://www.dwd.de/EN/ourservices/specialevents/temperature/20120315_cold_europe_february_2012_en.html (last access: 19 November 2021, 2012. a, b
Ehmele, F., Kautz, L.-A., Feldmann, H., and Pinto, J. G.: Long-term variance of heavy precipitation across central Europe using a large ensemble of regional climate model simulations, Earth Syst. Dynam., 11, 469–490, https://doi.org/10.5194/esd-11-469-2020, 2020. a
Ellis, F., Prince, H., Lovatt, G., and Whittington, R.: Mortality and morbidity in Birmingham during the 1976 heatwave, QJM-Int. J. Med., 49, 1–8, https://doi.org/10.1093/oxfordjournals.qjmed.a067600, 1980. a
Erekat, D. and Nofal, A.: Rapid damage, loss and needs assessment of Winter Storm Alexa: a report by the Palestinian authority, World Bank, Washington, DC, https://openknowledge.worldbank.org/handle/10986/20120 (last access: 20 November 2021), 2013. a
Ferranti, L., Corti, S., and Janousek, M.: Flow-dependent verification of the ECMWF ensemble over the Euro-Atlantic sector, Q. J. Roy. Meteor. Soc., 141, 916–924, https://doi.org/10.1002/qj.2411, 2015. a
Ferranti, L., Magnusson, L., Vitart, F., and Richardson, D. S.: How far in advance can we predict changes in large-scale flow leading to severe cold conditions over Europe?, Q. J. Roy. Meteor. Soc., 144, 1788–1802, https://doi.org/10.1002/qj.3341, 2018. a, b, c, d
Ferranti, L., Magnusson, L., Vitart, F., and Richardson, D.: A new product to flag up the risk of cold spells in Europe weeks ahead, ECMWF Newsletter, 158, 15–20, 2019. a, b
Fink, A. H., Brücher, T., Krüger, A., Leckebusch, G. C., Pinto, J. G., and Ulbrich, U.: The 2003 European summer heatwaves and drought – synoptic diagnosis and impacts, Weather, 59, 209–216, https://doi.org/10.1256/wea.73.04, 2004. a, b, c, d
Fink, A. H., Brücher, T., Ermert, V., Krüger, A., and Pinto, J. G.: The European storm Kyrill in January 2007: synoptic evolution, meteorological impacts and some considerations with respect to climate change, Nat. Hazards Earth Syst. Sci., 9, 405–423, https://doi.org/10.5194/nhess-9-405-2009, 2009. a, b, c
Finlay, S. E., Moffat, A., Gazzard, R., Baker, D., and Murray, V.: Health impacts of wildfires, PLoS currents, 4, e4f959951cce2c, https://doi.org/10.1371/4f959951cce2c, 2012. a
Fischer, E. M. and Schär, C.: Consistent geographical patterns of changes in high-impact European heatwaves, Nat. Geosci., 3, 398–403, https://doi.org/10.1038/ngeo866, 2010. a
Fischer, E. M., Seneviratne, S. I., Vidale, P. L., Lüthi, D., and Schär, C.: Soil Moisture – Atmosphere Interactions during the 2003 European Summer Heat Wave, J. Climate, 20, 5081–5099, https://doi.org/10.1175/JCLI4288.1, 2007. a, b, c, d
Folland, C. K., Karl, T. R., and Jim Salinger, M.: Observed climate variability and change, Weather, 57, 269–278, https://doi.org/10.1256/004316502320517353, 2002. a
Forzieri, G., Cescatti, A., e Silva, F. B., and Feyen, L.: Increasing risk over time of weather-related hazards to the European population: a data-driven prognostic study, Lancet Planetary Health, 1, e200–e208, https://doi.org/10.1016/S2542-5196(17)30082-7, 2017. a
Fragkoulidis, G., Wirth, V., Bossmann, P., and Fink, A. H.: Linking Northern Hemisphere temperature extremes to Rossby wave packets, Q. J. Roy. Meteor. Soc., 144, 553–566, https://doi.org/10.1002/qj.3228, 2018. a
Francis, J. A. and Vavrus, S. J.: Evidence for a wavier jet stream in response to rapid Arctic warming, Environ. Res. Lett., 10, 014005, https://doi.org/10.1088/1748-9326/10/1/014005, 2015. a
Frederiksen, J. S., Collier, M. A., and Watkins, A. B.: Ensemble prediction of blocking regime transitions, Tellus A, 56, 485–500, https://doi.org/10.3402/tellusa.v56i5.14460, 2004. a, b
Froidevaux, P., Schwanbeck, J., Weingartner, R., Chevalier, C., and Martius, O.: Flood triggering in Switzerland: the role of daily to monthly preceding precipitation, Hydrol. Earth Syst. Sci., 19, 3903–3924, https://doi.org/10.5194/hess-19-3903-2015, 2015. a
Gabriel, A. and Peters, D.: A diagnostic study of different types of Rossby wave breaking events in the northern extratropics, J. Meteorol. Soc. Jpn., 86, 613–631, 2008. a
Garcia-Herrera, R. and Barriopedro, D.: Northern Hemisphere snow cover and atmospheric blocking variability, J. Geophys. Res.-Atmos., 111, D21104, https://doi.org/10.1029/2005jd006975, 2006. a, b
Garcia-Herrera, R., Hernández, E., Barriopedro, D., Paredes, D., Trigo, R. M., Trigo, I. F., and Mendes, M. A.: The outstanding 2004/05 drought in the Iberian Peninsula: associated atmospheric circulation, J. Hydrometeorol., 8, 483–498, https://doi.org/10.1175/JHM578.1, 2007. a, b, c
García-Herrera, R., Díaz, J., Trigo, R. M., Luterbacher, J., and Fischer, E. M.: A review of the European summer heat wave of 2003, Crit. Rev. Env. Sci. Tec., 40, 267–306, https://doi.org/10.1080/10643380802238137, 2010. a, b
García-Herrera, R., Garrido-Perez, J. M., Barriopedro, D., Ordóñez, C., Vicente-Serrano, S. M., Nieto, R., Gimeno, L., Sorí, R., and Yiou, P.: The European 2016/17 Drought, J. Climate, 32, 3169–3187, https://doi.org/10.1175/JCLI-D-18-0331.1, 2019. a, b, c
Gasparrini, A., Guo, Y., Hashizume, M., Lavigne, E., Zanobetti, A., Schwartz, J., Tobias, A., Tong, S., Rocklöv, J., Forsberg, B., Leone, M., De Sario, M., Bell, M. L., Guo, Y. L., Wu, C., Kan, H., Yi, S., Coelho, M. S. Z. S., Saldiva, P. H. N., Honda, Y., Kim, H., and Armstrong, B.: Mortality risk attributable to high and low ambient temperature: a multicountry observational study, Lancet, 386, 369–375, https://doi.org/10.1016/S0140-6736(14)62114-0, 2015. a, b
Glisan, J. M.: Two extreme cases of atmospheric blocking over Europe and North America, PhD thesis, University of Missouri–Columbia, https://doi.org/10.32469/10355/5094, 2007. a
Gollan, G. and Greatbatch, R. J.: The relationship between northern hemisphere winter blocking and tropical modes of variability, J. Climate, 30, 9321–9337, https://doi.org/10.1175/JCLI-D-16-0742.1, 2017. a
Grams, C. M., Binder, H., Pfahl, S., Piaget, N., and Wernli, H.: Atmospheric processes triggering the central European floods in June 2013, Nat. Hazards Earth Syst. Sci., 14, 1691–1702, https://doi.org/10.5194/nhess-14-1691-2014, 2014. a, b, c, d
Grams, C. M., Beerli, R., Pfenninger, S., Staffell, I., and Wernli, H.: Balancing Europe's wind-power output through spatial deployment informed by weather regimes, Nat. Clim. Change, 7, 557–562, https://doi.org/10.1038/nclimate3338, 2017. a
Green, J. S. A.: The weather during July 1976: Some dynamical considerations of the droughts, Weather, 32, 120–126, https://doi.org/10.1002/j.1477-8696.1977.tb04532.x, 1977. a, b
Grumm, R. H.: The Central European and Russian Heat Event of July–August 2010, B. Am. Meteorol. Soc., 92, 1285–1296, https://doi.org/10.1175/2011BAMS3174.1, 2011. a, b, c, d
Haigh, I. D., Wadey, M. P., Wahl, T., Ozsoy, O., Nicholls, R. J., Brown, J. M., Horsburgh, K., and Gouldby, B.: Spatial and temporal analysis of extreme sea level and storm surge events around the coastline of the UK, Scientific Data, 3, 1–14, https://doi.org/10.1038/sdata.2016.107, 2016. a
Haines, D. A.: A lower atmosphere severity index for wildlife fires, National Weather Digest, 13, 23–27, 1989. a
Hamill, T. M. and Kiladis, G. N.: Skill of the MJO and Northern Hemisphere blocking in GEFS medium-range reforecasts, Mon. Weather Rev., 142, 868–885, https://doi.org/10.1175/MWR-D-13-00199.1, 2014. a, b
Hauser, M., Orth, R., and Seneviratne, S. I.: Role of soil moisture versus recent climate change for the 2010 heat wave in western Russia, Geophys. Res. Lett., 43, 2819–2826, https://doi.org/10.1002/2016GL068036, 2016. a
Heim Jr., R. R.: A review of twentieth-century drought indices used in the United States, B. Am. Meteorol. Soc., 83, 1149–1166, https://doi.org/10.1175/1520-0477-83.8.1149, 2002. a
Henderson, S. A., Maloney, E. D., and Barnes, E. A.: The influence of the Madden–Julian oscillation on Northern Hemisphere winter blocking, J. Climate, 29, 4597–4616, https://doi.org/10.1175/JCLI-D-15-0502.1, 2016. a
Hénin, R., Ramos, A. M., Pinto, J. G., and Liberato, M. L.: A ranking of concurrent precipitation and wind events for the Iberian Peninsula, Int. J. Climatol., 41, 1421–1437, https://doi.org/10.1002/joc.6829, 2020. a, b
Hersbach, H., Bell, B., Berrisford, P., Biavati, G., Horányi, A., Muñoz Sabater, J., Nicolas, J., Peubey, C., Radu, R., Rozum, I., Schepers, D., Simmons, A., Soci, C., Dee, D., and Thépaut, J.-N.: ERA5 monthly averaged data on single levels from 1979 to present, Copernicus Climate Change Service (C3S) Climate Data Store (CDS) [data set], https://doi.org/10.24381/cds.f17050d7, 2019a. a
Hersbach, H., Bell, B., Berrisford, P., Biavati, G., Horányi, A., Muñoz Sabater, J., Nicolas, J., Peubey, C., Radu, R., Rozum, I., Schepers, D., Simmons, A., Soci, C., Dee, D., and Thépaut, J.-N.: ERA5 monthly averaged data on pressure levels from 1979 to present. Copernicus Climate Change Service (C3S) Climate Data Store (CDS) [data set], https://doi.org/10.24381/cds.6860a573, 2019b. a
Hersbach, H., Bell, B., Berrisford, P., Hirahara, S., Horányi, A., Muñoz-Sabater, J., Nicolas, J., Peubey, C., Radu, R., Schepers, D., Simmons, A., Soci, C., Abdalla, S., Abellan, X., Balsamo, G., Bechtold, P., Biavati, G., Bidlot, J., Bonavita, M., De Chiara, G., Dahlgren, P., Dee, D., Diamantakis, M., Dragani, R., Flemming, J., Forbes, R., Fuentes, M., Geer, A., Haimberger, L., Healy, S., Hogan, R. J., Hólm, E., Janisková, M., Keeley, S., Laloyaux, P., Lopez, P., Lupu, C., Radnoti, G., de Rosnay, P., Rozum, I., Vamborg, F., Villaume, S., and Thépaut, J.-N.: The ERA5 global reanalysis, Q. J. Roy. Meteor. Soc., 146, 1999–2049, https://doi.org/10.1002/qj.3803, 2020. a, b, c
Hofherr, T. and Kunz, M.: Extreme wind climatology of winter storms in Germany, Clim. Res., 41, 105–123, https://doi.org/10.3354/cr00844, 2010. a
Holbrook, N. J., Gupta, A. S., Oliver, E. C., Hobday, A. J., Benthuysen, J. A., Scannell, H. A., Smale, D. A., and Wernberg, T.: Keeping pace with marine heatwaves, Nature Reviews Earth & Environment, 1, 482–493, https://doi.org/10.1038/s43017-020-0068-4, 2020. a
Horsburgh, K. and Wilson, C.: Tide-surge interaction and its role in the distribution of surge residuals in the North Sea, J. Geophys. Res.-Oceans, 112, C08003, https://doi.org/10.1029/2006JC004033, 2007. a
Horton, D. E., Johnson, N. C., Singh, D., Swain, D. L., Rajaratnam, B., and Diffenbaugh, N. S.: Contribution of changes in atmospheric circulation patterns to extreme temperature trends, Nature, 522, 465–469, https://doi.org/10.1038/nature14550, 2015. a
Hoskins, B. J. and Sardeshmukh, P. D.: A diagnostic study of the dynamics of the northern hemisphere winter of 1985–86, Q. J. Roy. Meteor. Soc., 113, 759–778, https://doi.org/10.1002/qj.49711347705, 1987. a, b
Hoskins, B. J., McIntyre, M. E., and Robertson, A. W.: On the use and significance of isentropic potential vorticity maps, Q. J. Roy. Meteor. Soc., 111, 877–946, https://doi.org/10.1002/qj.49711147002, 1985. a, b, c, d
Huang, C. S. and Nakamura, N.: Local finite-amplitude wave activity as a diagnostic of anomalous weather events, J. Atmos. Sci., 73, 211–229, https://doi.org/10.1175/JAS-D-15-0194.1, 2016. a
Huguenin, M. F., Fischer, E. M., Kotlarski, S., Scherrer, S. C., Schwierz, C., and Knutti, R.: Lack of Change in the Projected Frequency and Persistence of Atmospheric Circulation Types Over Central Europe, Geophys. Res. Lett., 47, e2019GL086132, https://doi.org/10.1029/2019GL086132, 2020. a
Ionita, M., Dima, M., Lohmann, G., Scholz, P., and Rimbu, N.: Predicting the June 2013 European flooding based on precipitation, soil moisture, and sea level pressure, J. Hydrometeorol., 16, 598–614, https://doi.org/10.1175/JHM-D-14-0156.1, 2015. a
Irwin, J. F.: Raging rivers and propaganda weevils: transnational disaster relief, Cold War politics, and the 1954 Danube and Elbe floods, Diplomatic Hist., 40, 893–921, https://doi.org/10.1093/dh/dhv053, 2016. a
Janjić, J., Gallagher, S., and Dias, F.: Case study of the winter 2013/2014 extreme wave events off the west coast of Ireland, Adv. Sci. Res., 15, 145–157, https://doi.org/10.5194/asr-15-145-2018, 2018. a
Jensen, J., Arns, A., and Wahl, T.: Yet another 100yr storm surge event: the role of individual storm surges on design water levels, J. Mar. Sci. Technol., 23, 882–887, https://doi.org/10.6119/JMST-015-0610-5, 2015. a
Jézéquel, A., Bevacqua, E., d'Andrea, F., Thao, S., Vautard, R., Vrac, M., and Yiou, P.: Conditional and residual trends of singular hot days in Europe, Environ. Res. Lett., 15, 064018, https://doi.org/10.1088/1748-9326/ab76dd, 2020. a
Jung, T., Miller, M. J., Palmer, T. N., Towers, P., Wedi, N., Achuthavarier, D., Adams, J. M., Altshuler, E. L., Cash, B. A., Kinter Iii, J. L., Marx, L., Stan, C., and Hodges, K. I.: High-resolution global climate simulations with the ECMWF model in Project Athena: Experimental design, model climate, and seasonal forecast skill, J. Climate, 25, 3155–3172, https://doi.org/10.1175/JCLI-D-11-00265.1, 2012. a
Karpechko, A. Y., Charlton-Perez, A., Balmaseda, M., Tyrrell, N., and Vitart, F.: Predicting sudden stratospheric warming 2018 and its climate impacts with a multimodel ensemble, Geophys. Res. Lett., 45, 13–538, https://doi.org/10.1029/2018GL081091, 2018. a, b
Karremann, M. K., Liberato, M. L., Ordóñez, P., and Pinto, J. G.: Characterization of synoptic conditions and cyclones associated with top ranking potential wind loss events over Iberia, Atmos. Sci. Lett., 17, 354–361, https://doi.org/10.1002/asl.665, 2016. a
Kautz, L.-A., Polichtchouk, I., Birner, T., Garny, H., and Pinto, J. G.: Enhanced extended-range predictability of the 2018 late-winter Eurasian cold spell due to the stratosphere, Q. J. Roy. Meteor. Soc., 146, 1040–1055, https://doi.org/10.1002/qj.3724, 2020. a, b
Kelemen, F. D., Ludwig, P., Reyers, M., Ulbrich, S., and Pinto, J. G.: Evaluation of moisture sources for the Central European summer flood of May/June 2013 based on regional climate model simulations, Tellus A, 68, 29288, https://doi.org/10.3402/tellusa.v68.29288, 2016. a
Kennedy, D., Parker, T., Woollings, T., Harvey, B., and Shaffrey, L.: The response of high-impact blocking weather systems to climate change, Geophys. Res. Lett., 43, 7250–7258, https://doi.org/10.1002/2016GL069725, 2016. a, b
Kjellström, E., Nikulin, G., Hansson, U., Strandberg, G., and Ullerstig, A.: 21st century changes in the European climate: uncertainties derived from an ensemble of regional climate model simulations, Tellus A, 63, 24–40, https://doi.org/10.1111/j.1600-0870.2010.00475.x, 2011. a
Koppe, C., Kovasts, S., Jendritzky, G., and Menne, B.: Heat-waves: risks and responses, Tech. rep., vol. 2, Copenhagen: WHO Regional Office for Europe, ISBN 92 890 1094 0., 2004. a
Kornhuber, K., Coumou, D., Vogel, E., Lesk, C., Donges, J. F., and an Radley M. Horton, J. L.: Amplified Rossby waves enhance risk of concurrent heatwaves in major breadbasket regions, Nat. Clim. Change, 10, 48–53, https://doi.org/10.1038/s41558-019-0637-z, 2020. a, b
Kovats, R. S. and Kristie, L. E.: Heatwaves and public health in Europe, Eur. J. Public Health, 16, 592–599, https://doi.org/10.1093/eurpub/ckl049, 2006. a
Kron, W., Löw, P., and Kundzewicz, Z. W.: Changes in risk of extreme weather events in Europe, Environ. Sci. Pol., 100, 74–83, https://doi.org/10.1016/j.envsci.2019.06.007, 2019. a, b, c, d, e
Lassnig, E., Dinkhauser, P., Maurer, E., and Eber, B.: Life-threatening heat stroke presenting with ST elevations: a report of consecutive cases during the heat wave in Austria in July 2013, Wien. Klin. Wochenschr., 126, 491–494, https://doi.org/10.1007/s00508-014-0531-7, 2014. a
Lau, W. K. M. and Kim, K.-M.: The 2010 Pakistan Flood and Russian Heat Wave: Teleconnection of Hydrometeorological Extremes, J. Hydrometeorol., 13, 392–403, https://doi.org/10.1175/JHM-D-11-016.1, 2012. a, b, c, d, e, f
Lavaysse, C., Naumann, G., Alfieri, L., Salamon, P., and Vogt, J.: Predictability of the European heat and cold waves, Clim. Dynam., 52, 2481–2495, https://doi.org/10.1007/s00382-018-4273-5, 2019. a
Lejenäs, H.: The Severe Winter in Europe 1941–42: The Large-scale Circulation, Cut-off Lows, and Blocking, B. Am. Meteorol. Soc., 70, 271–281, https://doi.org/10.1175/1520-0477(1989)070<0271:TSWIET>2.0.CO;2, 1989. a, b
Lenggenhager, S. and Martius, O.: Atmospheric blocks modulate the odds of heavy precipitation events in Europe, Clim. Dynam., 53, 4155–4171, https://doi.org/10.1007/s00382-019-04779-0, 2019. a, b, c, d, e, f, g, h
Lenggenhager, S. and Martius, O.: Quantifying the link between heavy precipitation and Northern Hemisphere blocking – A Lagrangian analysis, Atmos. Sci. Lett., 21, 1–12, https://doi.org/10.1002/asl.999, 2020. a
Lenggenhager, S., Croci-Maspoli, M., Bronnimann, S., and Martius, O.: On the dynamical coupling between atmospheric blocks and heavy precipitation events: A discussion of the southern Alpine flood in October 2000, Q. J. Roy. Meteor. Soc., 145, 530–545, https://doi.org/10.1002/qj.3449, 2019. a, b, c, d, e, f, g, h
Leonard, M., Westra, S., Phatak, A., Lambert, M., van den Hurk, B., McInnes, K., Risbey, J., Schuster, S., Jakob, D., and Stafford-Smith, M.: A compound event framework for understanding extreme impacts, WIRES Climate Change, 5, 113–128, https://doi.org/10.1002/wcc.252, 2014. a
Lhotka, O. and Kysely, J.: Hot Central-European summer of 2013 in a long-term context, Int. J. Climatol., 35, 4399–4407, https://doi.org/10.1002/joc.4277, 2015. a, b
Li, R. K., Woollings, T., O'Reilly, C., and Scaife, A. A.: Effect of the North Pacific Tropospheric Waveguide on the Fidelity of Model El Niño Teleconnections, J. Climate, 33, 5223–5237, https://doi.org/10.1175/JCLI-D-19-0156.1, 2020. a
Liu, Q.: On the definition and persistence of blocking, Tellus A, 46, 286–298, https://doi.org/10.1034/j.1600-0870.1994.t01-2-00004.x, 1994. a, b
Luo, D., Cha, J., Zhong, L., and Dai, A.: A nonlinear multiscale interaction model for atmospheric blocking: The eddy-blocking matching mechanism, Q. J. Roy. Meteor. Soc., 140, 1785–1808, https://doi.org/10.1002/qj.2337, 2014. a, b
Luo, D., Xiao, Y., Yao, Y., Dai, A., Simmonds, I., and Franzke, C. L.: Impact of Ural blocking on winter warm Arctic–cold Eurasian anomalies. Part I: Blocking-induced amplification, J. Climate, 29, 3925–3947, https://doi.org/10.1175/JCLI-D-15-0611.1, 2016. a, b
Luo, D., Chen, X., Dai, A., and Simmonds, I.: Changes in atmospheric blocking circulations linked with winter Arctic warming: A new perspective, J. Climate, 31, 7661–7678, https://doi.org/10.1175/JCLI-D-18-0040.1, 2018. a
Luo, D. H., Yao, Y., Dai, A. G., and Feldstein, S. B.: The Positive North Atlantic Oscillation with Downstream Blocking and Middle East Snowstorms: The Large-Scale Environment, J. Climate, 28, 6398–6418, https://doi.org/10.1175/Jcli-D-15-0184.1, 2015. a, b, c, d
Lupo, A. R.: Atmospheric blocking events: a review, Ann. NY Acad. Sci., 1504, 5–24, https://doi.org/10.1111/nyas.14557, 2020. a
Lupo, A. R. and Smith, P. J.: Planetary and synoptic-scale interactions during the life cycle of a mid-latitude blocking anticyclone over the North Atlantic, Tellus A, 47, 575–596, https://doi.org/10.1034/j.1600-0870.1995.00106.x, 1995. a, b
Maddison, J., Gray, S., Martínez-Alvarado, O., and Williams, K.: Upstream cyclone influence on the predictability of block onsets over the Euro-Atlantic region, Mon. Weather Rev., 147, 1277–1296, https://doi.org/10.1175/MWR-D-18-0226.1, 2019. a
Maddison, J. W., Gray, S. L., Martínez-Alvarado, O., and Williams, K. D.: Impact of model upgrades on diabatic processes in extratropical cyclones and downstream forecast evolution, Q. J. Roy. Meteor. Soc., 146, 1322–1350, https://doi.org/10.1002/qj.3739, 2020. a
Madonna, E., Wernli, H., Joos, H., and Martius, O.: Warm conveyor belts in the ERA-Interim dataset (1979–2010). Part I: Climatology and potential vorticity evolution, J. Climate, 27, 3–26, https://doi.org/10.1175/JCLI-D-12-00720.1, 2014. a
Maher, N., Milinski, S., Suarez-Gutierrez, L., Botzet, M., Kornblueh, L., Takano, Y., Kröger, J., Ghosh, R., Hedemann, C., Li, C., Li, H., Manzini, E., Notz, D., Putrasahan, D., Boysen, L., Claussen, M., Ilyina, T., Olonscheck, D., Raddatz, T., Stevens, B., and Marotzke, J.: The Max Planck Institute Grand Ensemble: enabling the exploration of climate system variability, J. Adv. Model. Earth Syst., 11, 2050–2069, https://doi.org/10.1029/2019MS001639, 2019. a
Mailier, P. J., Stephenson, D. B., Ferro, C. A., and Hodges, K. I.: Serial clustering of extratropical cyclones, Mon. Weather Rev., 134, 2224–2240, https://doi.org/10.1175/MWR3160.1, 2006. a, b, c
Mann, M. E., Rahmstorf, S., Kornhuber, K., Steinman, B. A., Miller, S. K., Petri, S., and Coumou, D.: Projected changes in persistent extreme summer weather events: The role of quasi-resonant amplification, Sci. Adv., 4, eaat3272, https://doi.org/10.1126/sciadv.aat3272, 2018. a
Martineau, P., Chen, G., and Burrows, D. A.: Wave events: climatology, trends, and relationship to Northern Hemisphere winter blocking and weather extremes, J. Climate, 30, 5675–5697, https://doi.org/10.1175/JCLI-D-16-0692.1, 2017. a
Martínez-Alvarado, O., Maddison, J. W., Gray, S. L., and Williams, K. D.: Atmospheric blocking and upper-level Rossby-wave forecast skill dependence on model configuration, Q. J. Roy. Meteor. Soc., 144, 2165–2181, https://doi.org/10.1002/qj.3326, 2018. a
Masato, G., Hoskins, B., and Woollings, T. J.: Wave-breaking characteristics of midlatitude blocking, Q. J. Roy. Meteor. Soc., 138, 1285–1296, https://doi.org/10.1002/qj.990, 2012. a
Masato, G., Hoskins, B. J., and Woollings, T.: Wave-breaking characteristics of Northern Hemisphere winter blocking: A two-dimensional approach, J. Climate, 26, 4535–4549, https://doi.org/10.1175/JCLI-D-12-00240.1, 2013a. a, b
Masato, G., Hoskins, B. J., and Woollings, T.: Winter and summer Northern Hemisphere blocking in CMIP5 models, J. Climate, 26, 7044–7059, https://doi.org/10.1175/JCLI-D-12-00466.1, 2013b. a, b, c
Masih, I., Maskey, S., Mussá, F. E. F., and Trambauer, P.: A review of droughts on the African continent: a geospatial and long-term perspective, Hydrol. Earth Syst. Sci., 18, 3635–3649, https://doi.org/10.5194/hess-18-3635-2014, 2014. a
Matsueda, M.: Blocking predictability in operational medium-range ensemble forecasts, Sola, 5, 113–116, https://doi.org/10.2151/sola.2009-029, 2009. a, b
Matsueda, M.: Predictability of Euro-Russian blocking in summer of 2010, Geophys. Res. Lett., 38, L06801, https://doi.org/10.1029/2010GL046557, 2011. a, b
Matsueda, M. and Endo, H.: The robustness of future changes in Northern Hemisphere blocking: A large ensemble projection with multiple sea surface temperature patterns, Geophys. Res. Lett., 44, 5158–5166, https://doi.org/10.1002/2017GL073336, 2017. a
Matsueda, M. and Palmer, T.: Estimates of flow-dependent predictability of wintertime Euro-Atlantic weather regimes in medium-range forecasts, Q. J. Roy. Meteor. Soc., 144, 1012–1027, https://doi.org/10.1002/qj.3265, 2018. a
Matsueda, M., Mizuta, R., and Kusunoki, S.: Future change in wintertime atmospheric blocking simulated using a 20-km-mesh atmospheric global circulation model, J. Geophys. Res.-Atmos., 114, D12114, https://doi.org/10.1029/2009JD011919, 2009. a, b
Matthews, T., Murphy, C., Wilby, R. L., and Harrigan, S.: Stormiest winter on record for Ireland and UK, Nat. Clim. Change, 4, 738–740, https://doi.org/10.1038/nclimate2336, 2014. a
Meehl, G. A. and Tebaldi, C.: More Intense, More Frequent, and Longer Lasting Heat Waves in the 21st Century, Science, 305, 994–997, https://doi.org/10.1126/science.1098704, 2004. a, b
Merrifield, A. L., Simpson, I. R., McKinnon, K. A., Sippel, S., Xie, S.-P., and Deser, C.: Local and Nonlocal Land Surface Influence in European Heatwave Initial Condition Ensembles, Geophys. Res. Lett., 46, 14082–14092, https://doi.org/10.1029/2019GL083945, 2019. a
Merz, R. and Bloschl, G.: A process typology of regional floods, Water Resour. Res., 39, 1340, https://doi.org/10.1029/2002wr001952, 2003. a, b
Messori, G. and Caballero, R.: On double Rossby wave breaking in the North Atlantic, J. Geophys. Res.-Atmos., 120, 11–129, https://doi.org/10.1002/2015JD023854, 2015. a
Methven, J.: Potential vorticity in warm conveyor belt outflow, Q. J. Roy. Meteor. Soc., 141, 1065–1071, https://doi.org/10.1002/qj.2393, 2015. a
Miller, D. E. and Wang, Z.: Skillful seasonal prediction of Eurasian winter blocking and extreme temperature frequency, Geophys. Res. Lett., 46, 11530–11538, https://doi.org/10.1029/2019GL085035, 2019. a
Miralles, D. G., Teuling, A. J., van Heerwaarden, C. C., and de Arellano, J. V.-G.: Mega-heatwave temperatures due to combined soil desiccation and atmospheric heat accumulation, Nat. Geosci., 7, 345–349, https://doi.org/10.1038/ngeo2141, 2014. a, b, c, d, e, f, g
Miralles, D. G., Gentine, P., Seneviratne, S. I., and Teuling, A. J.: Land–atmospheric feedbacks during droughts and heatwaves: state of the science and current challenges, Ann. NY Acad. Sci., 1436, 19–35, https://doi.org/10.1111/nyas.13912, 2019. a
Mitchell, D., Heaviside, C., Vardoulakis, S., Huntingford, C., Masato, G., Guillod, B. P., Frumhoff, P., Bowery, A., Wallom, D., and Allen, M.: Attributing human mortality during extreme heat waves to anthropogenic climate change, Environ. Res. Lett., 11, 074006, https://doi.org/10.1088/1748-9326/11/7/074006, 2016. a
Mitchell, J. F., Lowe, J., Wood, R. A., and Vellinga, M.: Extreme events due to human-induced climate change, Philos. T. Roy. Soc. A, 364, 2117–2133, https://doi.org/10.1098/rsta.2006.1816, 2006. a
Mohleji, S. and Pielke Jr., R.: Reconciliation of trends in global and regional economic losses from weather events: 1980–2008, Nat. Hazards Rev., 15, 04014009, https://doi.org/10.1061/(ASCE)NH.1527-6996.0000141, 2014. a
Mohr, S., Wandel, J., Lenggenhager, S., and Martius, O.: Relationship between atmospheric blocking and warm-season thunderstorms over western and central Europe, Q. J. Roy. Meteor. Soc., 145, 3040–3056, https://doi.org/10.1002/qj.3603, 2019. a, b, c
Mohr, S., Wilhelm, J., Wandel, J., Kunz, M., Portmann, R., Punge, H. J., Schmidberger, M., Quinting, J. F., and Grams, C. M.: The role of large-scale dynamics in an exceptional sequence of severe thunderstorms in Europe May–June 2018, Weather Clim. Dynam., 1, 325–348, https://doi.org/10.5194/wcd-1-325-2020, 2020. a, b, c, d
Moore, R. W., Martius, O., and Spengler, T.: The modulation of the subtropical and extratropical atmosphere in the Pacific basin in response to the Madden–Julian oscillation, Mon. Weather Rev., 138, 2761–2779, https://doi.org/10.1175/2010MWR3194.1, 2010. a
Nabizadeh, E., Hassanzadeh, P., Yang, D., and Barnes, E. A.: Size of the atmospheric blocking events: Scaling law and response to climate change, Geophys. Res. Lett., 46, 13488–13499, https://doi.org/10.1029/2019GL084863, 2019. a, b
Nabizadeh, E., Lubis, S. W., and Hassanchanzadeh, P.: The 3D Structure of Northern Hemisphere Blocking Events: Climatology, Role of Moisture, and Response to Climate Change, J. Climate, 34, 9837–9860, https://doi.org/10.1175/JCLI-D-21-0141.1, 2021. a
Nakamura, H. and Wallace, J. M.: Observed Changes in Baroclinic Wave Activity during the Life Cycles of Low-Frequency Circulation Anomalies, J. Atmos. Sci., 47, 1100–1116, https://doi.org/10.1175/1520-0469(1990)047<1100:OCIBWA>2.0.CO;2, 1989. a
Nakamura, H., Nakamura, M., and Anderson, J. L.: The Role of High- and Low-Frequency Dynamics in Blocking Formation, Mon. Weather Rev., 125, 2074–2093, 1997. a, b
Nakamura, N. and Huang, C. S. Y.: Atmospheric blocking as a traffic jam in the jet stream, Science, 361, 42–47, https://doi.org/10.1126/science.aat0721, 2018. a, b, c, d
Namias, J.: Seasonal Persistence and Recurrence of European Blocking during 1958-1960, Tellus, 16, 394–407, https://doi.org/10.1111/j.2153-3490.1964.tb00176.x, 1964. a, b
Ogi, M., Yamazaki, K., and Tachibana, Y.: The summer northern annular mode and abnormal summer weather in 2003, Geophys. Res. Lett., 32, L04706, https://doi.org/10.1029/2004GL021528, 2005. a, b
Olita, A., Sorgente, R., Natale, S., Gaberšek, S., Ribotti, A., Bonanno, A., and Patti, B.: Effects of the 2003 European heatwave on the Central Mediterranean Sea: surface fluxes and the dynamical response, Ocean Sci., 3, 273–289, https://doi.org/10.5194/os-3-273-2007, 2007. a
O'Reilly, C., Woollings, T., Zanna, L., and Weisheimer, A.: The impact of tropical precipitation on summertime Euro-Atlantic circulation via a circumglobal wave train, J. Climate, 31, 6481–6504, https://doi.org/10.1175/JCLI-D-17-0451.1, 2018. a
O'Reilly, C. H., Minobe, S., and Kuwano-Yoshida, A.: The influence of the Gulf Stream on wintertime European blocking, Clim. Dynam., 47, 1545–1567, https://doi.org/10.1007/s00382-015-2919-0, 2016. a
Parker, T., Woollings, T., and Weisheimer, A.: Ensemble sensitivity analysis of Greenland blocking in medium-range forecasts, Q. J. Roy. Meteor. Soc., 144, 2358–2379, https://doi.org/10.1002/qj.3391, 2018. a, b
Pasquier, J. T., Pfahl, S., and Grams, C. M.: Modulation of Atmospheric River Occurrence and Associated Precipitation Extremes in the North Atlantic Region by European Weather Regimes, Geophys. Res. Lett., 46, 1014–1023, https://doi.org/10.1029/2018gl081194, 2019. a
Patterson, M., Bracegirdle, T., and Woollings, T.: Southern Hemisphere atmospheric blocking in CMIP5 and future changes in the Australia-New Zealand sector, Geophys. Res. Lett., 46, 9281–9290, https://doi.org/10.1029/2019GL083264, 2019. a
Pelly, J. L. and Hoskins, B. J.: A new perspective on blocking, J. Atmos. Sci., 60, 743–755, https://doi.org/10.1175/1520-0469(2003)060<0743:ANPOB>2.0.CO;2, 2003a. a, b, c, d
Pelly, J. L. and Hoskins, B. J.: How well does the ECMWF Ensemble Prediction System predict blocking?, Q. J. Roy. Meteor. Soc., 129, 1683–1702, https://doi.org/10.1256/qj.01.173, 2003b. a
Péré, J. C., Bessagnet, B., Mallet, M., Waquet, F., Chiapello, I., Minvielle, F., Pont, V., and Menut, L.: Direct radiative effect of the Russian wildfires and its impact on air temperature and atmospheric dynamics during August 2010, Atmos. Chem. Phys., 14, 1999–2013, https://doi.org/10.5194/acp-14-1999-2014, 2014. a
Perkins, S. E.: A review on the scientific understanding of heatwaves – Their measurement, driving mechanisms, and changes at the global scale, Atmos. Res., 164–165, 242–267, https://doi.org/10.1016/j.atmosres.2015.05.014, 2015. a
Perkins, S. E., Alexander, L. V., and Nairn, J. R.: Increasing frequency, intensity and duration of observed global heatwaves and warm spells, Geophys. Res. Lett., 39, L20714, https://doi.org/10.1029/2012GL053361, 2012. a
Petoukhov, V., Rahmstorf, S., Petri, S., and Schellnhuber, H. J.: Quasiresonant amplification of planetary waves and recent Northern Hemisphere weather extremes, P. Natl. Acad. Sci. USA, 110, 5336–5341, https://doi.org/10.1073/pnas.1222000110, 2013. a
Pfahl, S.: Characterising the relationship between weather extremes in Europe and synoptic circulation features, Nat. Hazards Earth Syst. Sci., 14, 1461–1475, https://doi.org/10.5194/nhess-14-1461-2014, 2014. a, b, c, d, e, f, g, h
Pfahl, S. and Wernli, H.: Quantifying the relevance of atmospheric blocking for co-located temperature extremes in the Northern Hemisphere on (sub-)daily time scales, Geophys. Res. Lett., 39, L12807, https://doi.org/10.1029/2012GL052261, 2012. a, b, c
Pfahl, S., Schwierz, C., Croci-Maspoli, M., Grams, C. M., and Wernli, H.: Importance of latent heat release in ascending air streams for atmospheric blocking, Nat. Geosci., 8, 610–614, https://doi.org/10.1038/NGEO2487, 2015. a
Pfister, C., Weingartner, R., and Luterbacher, J.: Hydrological winter droughts over the last 450 years in the Upper Rhine basin: a methodological approach, Hydrolog. Sci. J., 51, 966–985, https://doi.org/10.1623/hysj.51.5.966, 2006. a
Piaget, N., Froidevaux, P., Giannakaki, P., Gierth, F., Martius, O., Riemer, M., Wolf, G., and Grams, C. M.: Dynamics of a local Alpine flooding event in October 2011: moisture source and large-scale circulation, Q. J. Roy. Meteor. Soc., 141, 1922–1937, https://doi.org/10.1002/qj.2496, 2015. a, b, c, d, e, f
Pinto, J. G., Brücher, T., Fink, A. H., and Krüger, A.: Extraordinary snow accumulations over parts of central Europe during the winter of 2005/06 and weather-related hazards, Weather, 62, 16–21, https://doi.org/10.1002/wea.19, 2007. a, b
Pinto, J. G., Ulbrich, S., Parodi, A., Rudari, R., Boni, G., and Ulbrich, U.: Identification and ranking of extraordinary rainfall events over Northwest Italy: The role of Atlantic moisture, J. Geophys. Res.-Atmos., 118, 2085–2097, https://doi.org/10.1002/jgrd.50179, 2013. a
Pinto, J. G., Gómara, I., Masato, G., Dacre, H. F., Woollings, T., and Caballero, R.: Large-scale dynamics associated with clustering of extratropical cyclones affecting Western Europe, J. Geophys. Res.-Atmos., 119, 13–704, https://doi.org/10.1002/2014JD022305, 2014. a, b, c, d
Pithan, F., Shepherd, T. G., Zappa, G., and Sandu, I.: Climate model biases in jet streams, blocking and storm tracks resulting from missing orographic drag, Geophys. Res. Lett., 43, 7231–7240, https://doi.org/10.1002/2016GL069551, 2016. a
Planchon, O., Quénol, H., Irimia, L., and Patriche, C.: European cold wave during February 2012 and impacts in wine growing regions of Moldavia (Romania), Theor. Appl. Climatol., 120, 469–478, https://doi.org/10.1007/s00704-014-1191-2, 2015. a, b
Plavcova, E. and Kysely, J.: Projected evolution of circulation types and their temperatures over Central Europe in climate models, Theor. Appl. Climatol., 114, 625–634, https://doi.org/10.1007/s00704-013-0874-4, 2013. a
Pope, R., Butt, E., Chipperfield, M., Doherty, R., Fenech, S., Schmidt, A., Arnold, S., and Savage, N.: The impact of synoptic weather on UK surface ozone and implications for premature mortality, Environ. Res. Lett., 11, 124004, https://doi.org/10.1088/1748-9326/11/12/124004, 2016. a
Priestley, M. D., Pinto, J. G., Dacre, H. F., and Shaffrey, L. C.: The role of cyclone clustering during the stormy winter of 2013/2014, Weather, 72, 187–192, https://doi.org/10.1002/wea.3025, 2017. a, b, c
Quandt, L.-A., Keller, J. H., Martius, O., and Jones, S. C.: Forecast Variability of the Blocking System over Russia in Summer 2010 and Its Impact on Surface Conditions, Weather Forecast., 32, 61–82, https://doi.org/10.1175/WAF-D-16-0065.1, 2017. a, b, c, d, e, f, g
Quandt, L.-A., Keller, J. H., Martius, O., Pinto, J. G., and Jones, S. C.: Ensemble Sensitivity Analysis of the Blocking System over Russia in Summer 2010, Mon. Weather Rev., 147, 657–675, https://doi.org/10.1175/MWR-D-18-0252.1, 2019. a, b, c
Quesada, B., Vautard, R., Yiou, P., Hirschi, M., and Seneviratne, S. I.: Asymmetric European summer heat predictability from wet and dry southern winters and springs, Nat. Clim. Change, 2, 736–741, https://doi.org/10.1038/nclimate1536, 2012. a
Rahmstorf, S. and Coumou, D.: Increase of extreme events in a warming world, P. Natl. Acad. Sci. USA, 108, 17905–17909, https://doi.org/10.1073/pnas.1101766108, 2011. a
Rahmstorf, S., Foster, G., and Cazenave, A.: Comparing climate projections to observations up to 2011, Environ. Res. Lett., 7, 044035, https://doi.org/10.1088/1748-9326/7/4/044035, 2012. a
Raška, P.: Flood risk perception in Central-Eastern European members states of the EU: a review, Nat. Hazards, 79, 2163–2179, https://doi.org/10.1007/s11069-015-1929-x, 2015. a
Rex, D. F.: Blocking action in the middle troposphere and its effect upon regional climate, Tellus, 2, 275–301, https://doi.org/10.1111/j.2153-3490.1950.tb00339.x, 1950. a, b, c
Reynolds, D. D., Lupo, A. R., Jensen, A. D., and Market, P. S.: The Predictability of Blocking Character in the Northern Hemisphere Using an Ensemble Forecast System, Open Atmospheric Science Journal, 13, 3–17, https://doi.org/10.2174/1874282301913010013, 2019. a
Rimbu, N., Stefan, S., Busuioc, A., and Georgescu, F.: Links between blocking circulation and precipitation extremes over Romania in summer, Int. J. Climatol., 36, 369–376, https://doi.org/10.1002/joc.4353, 2016. a, b
Robine, J.-M., Cheung, S. L. K., Le Roy, S., Van Oyen, H., Griffiths, C., Michel, J.-P., and Herrmann, F. R.: Death toll exceeded 70 000 in Europe during the summer of 2003, C. R. Biol., 331, 171–178, https://doi.org/10.1016/j.crvi.2007.12.001, 2008. a
Robinson, P. J.: On the definition of a heat wave, J. Appl. Meteorol., 40, 762–775, https://doi.org/10.1175/1520-0450(2001)040<0762:OTDOAH>2.0.CO;2, 2001. a
Rodrigues, R. R., Taschetto, A. S., Gupta, A. S., and Foltz, G. R.: Common cause for severe droughts in South America and marine heatwaves in the South Atlantic, Nat. Geosci., 12, 620–626, https://doi.org/10.1038/s41561-019-0393-8, 2019. a
Rodwell, M. J., Magnusson, L., Bauer, P., Bechtold, P., Bonavita, M., Cardinali, C., Diamantakis, M., Earnshaw, P., Garcia-Mendez, A., Isaksen, L., Källén, E., Klocke, D., Lopez, P., McNally, T., Persson, A., Prates, F., and Wedi, N.: Characteristics of occasional poor medium-range weather forecasts for Europe, B. Am. Meteorol. Soc., 94, 1393–1405, https://doi.org/10.1175/BAMS-D-12-00099.1, 2013. a
Rohrer, M., Brönnimann, S., Martius, O., Raible, C. C., and Wild, M.: Decadal variations of blocking and storm tracks in centennial reanalyses, Tellus A, 71, 1586236, https://doi.org/10.1080/16000870.2019.1586236, 2019. a, b, c
Röthlisberger, M. and Martius, O.: Quantifying the Local Effect of Northern Hemisphere Atmospheric Blocks on the Persistence of Summer Hot and Dry Spells, Geophys. Res. Lett., 46, 10101–10111, https://doi.org/10.1029/2019GL083745, 2019. a, b
Röthlisberger, M., Pfahl, S., and Martius, O.: Regional-scale jet waviness modulates the occurrence of midlatitude weather extremes, Geophys. Res. Lett., 43, 10989–10997, https://doi.org/10.1002/2016GL070944, 2016. a
Röthlisberger, M., Frossard, L., Bosart, L. F., Keyser, D., and Martius, O.: Recurrent Synoptic-Scale Rossby Wave Patterns and Their Effect on the Persistence of Cold and Hot Spells, J. Climate, 32, 3207–3226, https://doi.org/10.1175/JCLI-D-18-0664.1, 2019. a
Rucińska, D.: Describing Storm Xaver in disaster terms, Int. J. Disast. Risk Re., 34, 147–153, https://doi.org/10.1016/j.ijdrr.2018.11.012, 2019. a
Sánchez-Benítez, A., García-Herrera, R., Barriopedro, D., Sousa, P. M., and Trigo, R. M.: June 2017: the earliest European summer mega-heatwave of Reanalysis Period, Geophys. Res. Lett., 45, 1955–1962, https://doi.org/10.1002/2018GL077253, 2018. a
Santos, J. A., Pinto, J. G., and Ulbrich, U.: On the development of strong ridge episodes over the eastern North Atlantic, Geophys. Res. Lett., 36, L17804, https://doi.org/10.1029/2009GL039086, 2009. a, b
Santos, J. A., Woollings, T., and Pinto, J. G.: Are the winters 2010 and 2012 archetypes exhibiting extreme opposite behavior of the North Atlantic jet stream?, Mon. Weather Rev., 141, 3626–3640, https://doi.org/10.1175/MWR-D-13-00024.1, 2013. a, b, c, d, e
Santos, J. A., Pfahl, S., Pinto, J. G., and Wernli, H.: Mechanisms underlying temperature extremes in Iberia: a Lagrangian perspective, Tellus A, 67, 26032, https://doi.org/10.3402/tellusa.v67.26032, 2015. a, b
Scaife, A. A., Arribas, A., Blockley, E., Brookshaw, A., Clark, R. T., Dunstone, N., Eade, R., Fereday, D., Folland, C. K., Gordon, M., Hermanson, L., Knight, J. R., Lea, D. J., MacLachlan, C., Maidens, A., Martin, M., Peterson, A. K., Smith, D., Vellinga, M., Wallace, E., Waters, J., and Williams, A.: Skillful long-range prediction of European and North American winters, Geophys. Res. Lett., 41, 2514–2519, https://doi.org/10.1002/2014GL059637, 2014. a
Schalge, B., Blender, R., and Fraedrich, K.: Blocking detection based on synoptic filters, Adv. Meteorol., 2011, 717812, https://doi.org/10.1155/2011/717812, 2011. a
Schaller, N., Sillmann, J., Anstey, J., Fischer, E. M., Grams, C. M., and Russo, S.: Influence of blocking on Northern European and Western Russian heatwaves in large climate model ensembles, Environ. Res. Lett., 13, 054015, https://doi.org/10.1088/1748-9326/aaba55, 2018. a, b, c, d
Schär, C., Vidale, P. L., Lüthi, D., Frei, C., Häberli, C., Liniger, M. A., and Appenzeller, C.: The role of increasing temperature variability in European summer heatwaves, Nature, 427, 332–336, https://doi.org/10.1038/nature02300, 2004. a
Scherrer, S. C. and Appenzeller, C.: Swiss Alpine snow pack variability: major patterns and links to local climate and large-scale flow, Clim. Res., 32, 187–199, https://doi.org/10.3354/cr032187, 2006. a
Scherrer, S. C., Croci-Maspoli, M., Schwierz, C., and Appenzeller, C.: Two-dimensional indices of atmospheric blocking and their statistical relationship with winter climate patterns in the Euro-Atlantic region, Int. J. Climatol., 26, 233–249, https://doi.org/10.1002/joc.1250, 2006. a
Schiemann, R., Demory, M.-E., Shaffrey, L. C., Strachan, J., Vidale, P. L., Mizielinski, M. S., Roberts, M. J., Matsueda, M., Wehner, M. F., and Jung, T.: The resolution sensitivity of Northern Hemisphere blocking in four 25-km atmospheric global circulation models, J. Climate, 30, 337–358, https://doi.org/10.1175/JCLI-D-16-0100.1, 2017. a
Schneidereit, A., Schubert, S., Vargin, P., Lunkeit, F., Zhu, X., Peters, D. H. W., and Fraedrich, K.: Large-Scale Flow and the Long-Lasting Blocking High over Russia: Summer 2010, Mon. Weather Rev., 140, 2967–2981, https://doi.org/10.1175/MWR-D-11-00249.1, 2012. a, b
Schumacher, D. L., Keune, J., Van Heerwaarden, C. C., de Arellano, J. V.-G., Teuling, A. J., and Miralles, D. G.: Amplification of mega-heatwaves through heat torrents fuelled by upwind drought, Nat. Geosci., 12, 712–717, https://doi.org/10.1038/s41561-019-0431-6, 2019. a, b, c, d, e
Schwierz, C., Croci-Maspoli, M., and Davies, H.: Perspicacious indicators of atmospheric blocking, Geophys. Res. Lett., 31, L06125, https://doi.org/10.1029/2003GL019341, 2004. a, b
Screen, J. A.: Arctic amplification decreases temperature variance in northern mid-to high-latitudes, Nat. Clim. Change, 4, 577–582, https://doi.org/10.1038/nclimate2268, 2014. a
Seager, R., Kushnir, Y., Nakamura, J., Ting, M., and Naik, N.: Northern Hemisphere winter snow anomalies: ENSO, NAO and the winter of 2009/10, Geophys. Res. Lett., 37, L14703, https://doi.org/10.1029/2010GL043830, 2010. a, b, c
Seneviratne, S. I., Corti, T., Davin, E. L., Hirschi, M., Jaeger, E. B., Lehner, I., Orlowsky, B., and Teuling, A. J.: Investigating soil moisture–climate interactions in a changing climate: A review, Earth-Sci. Rev., 99, 125–161, https://doi.org/10.1016/j.earscirev.2010.02.004, 2010. a
Ser-Giacomi, E., Vasile, R., Recuerda, I., Hernández-García, E., and López, C.: Dominant transport pathways in an atmospheric blocking event, Chaos, 25, 087413, https://doi.org/10.1063/1.4928704, 2015. a
Shabbar, A., Huang, J., and Higuchi, K.: The relationship between the wintertime North Atlantic Oscillation and blocking episodes in the North Atlantic, Int. J. Climatol., 21, 355–369, https://doi.org/10.1002/joc.612, 2001. a, b, c
Shutts, G.: The propagation of eddies in diffluent jetstreams: Eddy vorticity forcing of “blocking” flow fields, Q. J. Roy. Meteor. Soc., 109, 737–761, https://doi.org/10.1002/qj.49710946204, 1983. a, b, c, d
Sillmann, J. and Croci-Maspoli, M.: Present and future atmospheric blocking and its impact on European mean and extreme climate, Geophys. Res. Lett., 36, L10702, https://doi.org/10.1029/2009GL038259, 2009. a, b
Sillmann, J., Croci-Maspoli, M., Kallache, M., and Katz, R. W.: Extreme cold winter temperatures in Europe under the influence of North Atlantic atmospheric blocking, J. Climate, 24, 5899–5913, https://doi.org/10.1175/2011JCLI4075.1, 2011. a, b, c
Sousa, P. M., Trigo, R. M., Barriopedro, D., Soares, P. M. M., Ramos, A. M., and Liberato, M. L. R.: Responses of European precipitation distributions and regimes to different blocking locations, Clim. Dynam., 48, 1141–1160, https://doi.org/10.1007/s00382-016-3132-5, 2017. a, b, c, d, e, f, g, h, i, j
Sousa, P. M., Trigo, R. M., Barriopedro, D., Soares, P. M. M., and Santos, J. A.: European temperature responses to blocking and ridge regional patterns, Clim. Dynam., 50, 457–477, https://doi.org/10.1007/s00382-017-3620-2, 2018. a, b, c, d, e, f, g
Sousa, P. M., Barriopedro, D., García-Herrera, R., Ordóñez, C., Soares, P. M., and Trigo, R. M.: Distinct influences of large-scale circulation and regional feedbacks in two exceptional 2019 European heatwaves, Communications Earth & Environment, 1, 1–13, https://doi.org/10.1038/s43247-020-00048-9, 2020. a
Sousa, P. M., Barriopedro, D., García-Herrera, R., Woollings, T., and Trigo, R. M.: A new combined detection algorithm for blocking and subtropical ridges, J. Climate, 34, 7735–7758, https://doi.org/10.1175/JCLI-D-20-0658.1, 2021. a, b, c, d, e, f, g
Sparks, T. H. and Menzel, A.: Observed changes in seasons: an overview, Int. J. Climatol., 22, 1715–1725, https://doi.org/10.1002/joc.821, 2002. a
Sparnocchia, S., Schiano, M. E., Picco, P., Bozzano, R., and Cappelletti, A.: The anomalous warming of summer 2003 in the surface layer of the Central Ligurian Sea (Western Mediterranean), Ann. Geophys., 24, 443–452, https://doi.org/10.5194/angeo-24-443-2006, 2006. a
Spensberger, C., Madonna, E., Boettcher, M., Grams, C. M., Papritz, L., Quinting, J. F., Röthlisberger, M., Sprenger, M., and Zschenderlein, P.: Dynamics of concurrent and sequential Central European and Scandinavian heatwaves, Q. J. Roy. Meteor. Soc., 146, 2998–3013, https://doi.org/10.1002/qj.3822, 2020. a, b
Staneva, J., Wahle, K., Koch, W., Behrens, A., Fenoglio-Marc, L., and Stanev, E. V.: Coastal flooding: impact of waves on storm surge during extremes – a case study for the German Bight, Nat. Hazards Earth Syst. Sci., 16, 2373–2389, https://doi.org/10.5194/nhess-16-2373-2016, 2016. a, b
Stefanon, M., D'Andrea, F., and Drobinski, P.: Heatwave classification over Europe and the Mediterranean region, Environ. Res. Lett., 7, 014023, https://doi.org/10.1088/1748-9326/7/1/014023, 2012. a
Steinfeld, D. and Pfahl, S.: The role of latent heating in atmospheric blocking dynamics: a global climatology, Clim. Dynam., 53, 6159–6180, https://doi.org/10.1007/s00382-019-04919-6, 2019. a, b
Steinfeld, D., Boettcher, M., Forbes, R., and Pfahl, S.: The sensitivity of atmospheric blocking to upstream latent heating – numerical experiments, Weather Clim. Dynam., 1, 405–426, https://doi.org/10.5194/wcd-1-405-2020, 2020. a
Stott, P. A., Christidis, N., Otto, F. E. L., Sun, Y., Vanderlinden, J.-P., van Oldenborgh, G. J., Vautard, R., von Storch, H., Walton, P., Yiou, P., and Zwiers, F. W.: Attribution of extreme weather and climate-related events, WIRES Climate Change, 7, 23–41, https://doi.org/10.1002/wcc.380, 2016. a
Stucki, P., Rickli, R., Bronnimann, S., Martius, O., Wanner, H., Grebner, D., and Luterbacher, J.: Weather patterns and hydro-climatological precursors of extreme floods in Switzerland since 1868, Meteorol. Z., 21, 531–550, https://doi.org/10.1127/0941-2948/2012/368, 2012. a, b, c
Stucki, P., Bandhauer, M., Heikkilä, U., Rössler, O., Zappa, M., Pfister, L., Salvisberg, M., Froidevaux, P., Martius, O., Panziera, L., and Brönnimann, S.: Reconstruction and simulation of an extreme flood event in the Lago Maggiore catchment in 1868, Nat. Hazards Earth Syst. Sci., 18, 2717–2739, https://doi.org/10.5194/nhess-18-2717-2018, 2018. a
Swain, D. L., Singh, D., Touma, D., and Diffenbaugh, N. S.: Attributing extreme events to climate change: A new frontier in a warming world, One Earth, 2, 522–527, https://doi.org/10.1016/j.oneear.2020.05.011, 2020. a
Swanson, K. L.: Dynamical aspects of extratropical tropospheric low-frequency variability, J. Climate, 15, 2145–2162, https://doi.org/10.1175/1520-0442(2002)015<2145:DAOETL>2.0.CO;2, 2002. a
Tayanc, M., Karaca, M., and Dalfes, H. N.: March 1987 cyclone (blizzard) over the eastern Mediterranean and Balkan region associated with blocking, Mon. Weather Rev., 126, 3036–3047, https://doi.org/10.1175/1520-0493(1998)126<3036:Mcbote>2.0.Co;2, 1998. a, b, c
Tibaldi, S. and Molteni, F.: On the operational predictability of blocking, Tellus A, 42, 343–365, https://doi.org/10.3402/tellusa.v42i3.11882, 1990. a, b, c
Tomczyk, A. M. and Bednorz, E.: Heat waves in Central Europe and their circulation conditions, Int. J. Climatol., 36, 770–782, https://doi.org/10.1002/joc.4381, 2016. a
Tramblay, Y., Koutroulis, A., Samaniego, L., Vicente-Serrano, V., Volaire, F., Boone, A., Le Page, M., Llasat, M. C., Albergel, C., Burak, S., Cailleret, M., Kalic, K., Dupuy, J.-L., Greve, P., Grillakis, M., Hanich, L., Jarlan, L., Martin, N., Martinez-Vilalta, J., Mouillot, F., Pulido-Velazquez, D., Quintana-Segui, P., Renard, D., Turco, M., Türkes, M., Trigo, R., Vidal, J.-P., and Villagrosa, R.: Challenges for drought assessment in the Mediterranean region under future climate scenarios, Earth-Sci. Rev., 210, 103348, https://doi.org/10.1016/j.earscirev.2020.103348, 2020. a
Trenberth, K. E. and Fasullo, J. T.: Climate extremes and climate change: The Russian heat wave and other climate extremes of 2010, J. Geophys. Res., 117, D17103, https://doi.org/10.1029/2012JD018020, 2012. a, b
Trigo, R. M., Trigo, I. F., DaCamara, C. C., and Osborn, T. J.: Climate impact of the European winter blocking episodes from the NCEP/NCAR Reanalyses, Clim. Dynam., 23, 17–28, https://doi.org/10.1007/s00382-004-0410-4, 2004. a, b, c, d
Tung, K. K. and Lindzen, R.: A theory of stationary long waves. Part I: A simple theory of blocking, Mon. Weather Rev., 107, 714–734, https://doi.org/10.1175/1520-0493(1979)107<0714:ATOSLW>2.0.CO;2, 1979. a
Tyrlis, E. and Hoskins, B.: Aspects of a Northern Hemisphere atmospheric blocking climatology, J. Atmos. Sci., 65, 1638–1652, https://doi.org/10.1175/2007JAS2337.1, 2008. a, b, c
van Garderen, L., Feser, F., and Shepherd, T. G.: A methodology for attributing the role of climate change in extreme events: a global spectrally nudged storyline, Nat. Hazards Earth Syst. Sci., 21, 171–186, https://doi.org/10.5194/nhess-21-171-2021, 2021. a
Vieno, M., Dore, A. J., Stevenson, D. S., Doherty, R., Heal, M. R., Reis, S., Hallsworth, S., Tarrason, L., Wind, P., Fowler, D., Simpson, D., and Sutton, M. A.: Modelling surface ozone during the 2003 heat-wave in the UK, Atmos. Chem. Phys., 10, 7963–7978, https://doi.org/10.5194/acp-10-7963-2010, 2010. a
Vitart, F., Balsamo, G., Buizza, R., Ferranti, L., Keeley, S., Magnusson, L., Molenti, F., and Weisheimer, A.: Sub-seasonal predictions, European Centre for Medium-Range Weather Forecasts, https://www.ecmwf.int/sites/default/files/elibrary/2014/12943-sub-seasonal-predictions.pdf (last access: 20 June 2021), 2014. a
Wachowicz, L. J., Preece, J. R., Mote, T. L., Barrett, B. S., and Henderson, G. R.: Historical trends of seasonal Greenland blocking under different blocking metrics, Int. J. Climatol., 41, E3263–E3278, https://doi.org/10.1002/joc.6923, 2021. a
Wang, C., Liu, H., and Lee, S.-K.: The record-breaking cold temperatures during the winter of 2009/2010 in the Northern Hemisphere, Atmos. Sci. Lett., 11, 161–168, https://doi.org/10.1002/asl.278, 2010. a, b, c
Wang, L. and Kuang, Z.: Evidence against a general positive eddy feedback in atmospheric blocking, arXiv [preprint], arXiv:1907.00999, 1 July 2019. a
Wanner, H., Brönnimann, S., Casty, C., Gyalistras, D., Luterbacher, J., Schmutz, C., Stephenson, D. B., and Xoplaki, E.: North Atlantic Oscillation–concepts and studies, Surv. Geophys., 22, 321–381, https://doi.org/10.1023/A:1014217317898, 2001. a
Webber, C. P., Dacre, H. F., Collins, W. J., and Masato, G.: The dynamical impact of Rossby wave breaking upon UK PM10 concentration, Atmos. Chem. Phys., 17, 867–881, https://doi.org/10.5194/acp-17-867-2017, 2017. a
Weber, J., Reyers, M., Beck, C., Timme, M., Pinto, J. G., Witthaut, D., and Schäfer, B.: Wind power persistence characterized by Superstatistics, Sci. Rep., 9, 1–15, https://doi.org/10.1038/s41598-019-56286-1, 2019. a, b
Webster, P., Toma, V. E., and Kim, H.-M.: Were the 2010 Pakistan floods predictable?, Geophys. Res. Lett., 38, L04806, https://doi.org/10.1029/2010GL046346, 2011. a
Weijenborg, C., de Vries, H., and Haarsma, R. J.: On the direction of Rossby wave breaking in blocking, Clim. Dynam., 39, 2823–2831, https://doi.org/10.1007/s00382-012-1332-1, 2012. a
Whan, K., Zwiers, F., and Sillmann, J.: The influence of atmospheric blocking on extreme winter minimum temperatures in North America, J. Climate, 29, 4361–4381, https://doi.org/10.1175/JCLI-D-15-0493.1, 2016. a
Witte, J. C., Douglass, A. R., da Silva, A., Torres, O., Levy, R., and Duncan, B. N.: NASA A-Train and Terra observations of the 2010 Russian wildfires, Atmos. Chem. Phys., 11, 9287–9301, https://doi.org/10.5194/acp-11-9287-2011, 2011. a
WMO: Assessment of the Observed Extreme Conditions during Late Boreal Winter 2011/2012, WCDMP 80. Publications Board: Geneva, Switzerland, https://library.wmo.int/index.php?lvl=notice_display&id=14504#.YZqCRk6ZOUk (last access: 19 June 2021), 2015. a
Woollings, T., Hoskins, B., Blackburn, M., and Berrisford, P.: A new Rossby wave–breaking interpretation of the North Atlantic Oscillation, J. Atmos. Sci., 65, 609–626, https://doi.org/10.1175/2007JAS2347.1, 2008. a
Woollings, T., Charlton-Perez, A., Ineson, S., Marshall, A., and Masato, G.: Associations between stratospheric variability and tropospheric blocking, J. Geophys. Res.-Atmos., 115, D06108, https://doi.org/10.1029/2009JD012742, 2010. a
Woollings, T., Barriopedro, D., Methven, J., Son, S.-W., Martius, O., Harvey, B., Sillmann, J., Lupo, A. R., and Seneviratne, S.: Blocking and its response to climate change, Current climate change reports, 4, 287–300, https://doi.org/10.1007/s40641-018-0108-z, 2018. a, b, c, d, e, f
Wulff, C. O. and Domeisen, D. I. V.: Higher Subseasonal Predictability of Extreme Hot European Summer Temperatures as Compared to Average Summers, Geophys. Res. Lett., 46, 11520–11529, https://doi.org/10.1029/2019GL084314, 2019. a
Yao, Y. and De-Hai, L.: The Anomalous European Climates Linked to Different Euro-Atlantic Blocking, Atmos. Ocean. Sci. Lett., 7, 309–313, https://doi.org/10.3878/j.issn.1674-2834.14.0001, 2014. a, b
Yessimbet, K., Ossó, A., Kaltenberger, R., Magnusson, L., and Steiner, A.: Heavy Alpine snowfall in January 2019 connected to atmospheric blocking, Weather, 77, 7–15, https://doi.org/10.1002/wea.4020, 2022. a, b
Zscheischler, J., Westra, S., van den Hurk, B. J. J. M., Seneviratne, S. I., Ward, P. J., Pitman, A., AghaKouchak, A., Bresch, D. N., Leonard, M., Wahl, T., and Zhang, X.: Future climate risk from compound events, Nat. Clim. Change, 8, 469–477, https://doi.org/10.1038/s41558-018-0156-3, 2018. a
Zscheischler, J., Martius, O., Westra, S., Bevacqua, E., Raymond, C., Horton, R. M., van den Hurk, B., AghaKouchak, A., Jézéquel, A., Mahecha, M. D., Maraun, D., Ramos, A. M., Ridder, N. N., Thiery, W., and Vignotto, E.: A typology of compound weather and climate events, Nature Reviews Earth & Environment, 1, 333–347, https://doi.org/10.1038/s43017-020-0060-z, 2020. a, b, c
Zschenderlein, P., Fragkoulidis, G., Fink, A. H., and Wirth, V.: Large-scale Rossby wave and synoptic-scale dynamic analyses of the unusually late 2016 heatwave over Europe, Weather, 73, 275–283, https://doi.org/10.1002/wea.3278, 2018. a
Zschenderlein, P., Fink, A. H., Pfahl, S., and Wernli, H.: Processes determining heat waves across different European climates, Q. J. Roy. Meteor. Soc., 145, 2973–2989, https://doi.org/10.1002/qj.3599, 2019. a, b
Zschenderlein, P., Pfahl, S., Wernli, H., and Fink, A. H.: A Lagrangian analysis of upper-tropospheric anticyclones associated with heat waves in Europe, Weather Clim. Dynam., 1, 191–206, https://doi.org/10.5194/wcd-1-191-2020, 2020. a
- Abstract
- Introduction
- Atmospheric blocking
- Temperature extremes
- Hydrological extremes
- Other extremes
- The impact of atmospheric blocking on the predictability of extreme weather events
- The relation between atmospheric blocking and extreme weather events in the context of climate change
- Outlook and research perspectives
- Data availability
- Author contributions
- Competing interests
- Disclaimer
- Acknowledgements
- Financial support
- Review statement
- References
- Abstract
- Introduction
- Atmospheric blocking
- Temperature extremes
- Hydrological extremes
- Other extremes
- The impact of atmospheric blocking on the predictability of extreme weather events
- The relation between atmospheric blocking and extreme weather events in the context of climate change
- Outlook and research perspectives
- Data availability
- Author contributions
- Competing interests
- Disclaimer
- Acknowledgements
- Financial support
- Review statement
- References